3
Pharmacokinetics of Fluoride
This chapter updates pharmacokinetic information on fluoride developed since the earlier National Research Council review (NRC 1993). Particular attention is given to several potentially important issues for evaluation of the U.S. Environmental Protection Agency (EPA) maximum-contaminant-level goal (MCLG), including the accumulation of fluoride in bone, pharmacokinetic modeling, cross-species extrapolation, and susceptible populations. Consideration of biomarkers is provided in Chapter 2.
OVERVIEW OF FLUORIDE CHEMISTRY, UNITS, AND MEASUREMENT
Fluoride is the ionic form of fluorine, the most electronegative element. Water in the United States is typically fluoridated with fluorosilicates or sodium fluoride. In water at approximately neutral pH, fluorosilicates appear to entirely dissociate, producing fluoride ion, hydrofluoric acid (HF), and silicic acid (Si(OH)4). Fluoride reversibly forms HF in water. It also complexes with aluminum. See Chapter 2 for additional discussion of fluorosilicates and aluminum fluoride complexes.
Inorganic fluoride takes two primary forms in body fluids: fluoride ion and HF. Organofluorine compounds, and their potential relationship to inorganic fluoride, are discussed in Chapter 2 and later in this chapter.
A number of different units are commonly used to measure fluoride concentrations in water and biological samples (Table 3-1). Because the atomic weight of fluorine is 19, 1 µmol/L is equal to 0.019 milligrams per liter (mg/L). Bone ash is typically about 56% of wet bone by weight (Rao
TABLE 3-1 Commonly Used Units for Measuring Fluoride
Medium |
Unit |
Equivalent |
Water |
1 ppm |
1 mg/L |
Plasma |
1 µmol/L |
0.019 mg/L |
Bone ash |
1 ppm |
1 mg/kg |
et al. 1995), so 1,000 milligrams per kilogram (mg/kg) of fluoride in bone ash is equivalent to about 560 mg/kg wet weight.
Fluoride concentrations in body fluids typically are measured with a fluoride-specific electrode, an instrument that cannot reliably measure concentrations below about 0.019 mg/L and tends to overpredict at lower concentrations. As many people living in areas with artificially fluoridated water have plasma concentrations in this range, studies that rely on fluoride electrodes alone might tend to overpredict concentrations in plasma and body fluids. The hexamethyldisiloxane diffusion method provides a way around this problem by concentrating the fluoride in samples before analysis (reviewed by Whitford 1996).
SHORT REVIEW OF FLUORIDE PHARMACOKINETICS: ABSORPTION, DISTRIBUTION, AND ELIMINATION
A comprehensive review of fluoride pharmacokinetics is provided by Whitford (1996), and this section presents a brief overview of that information. The pharmacokinetics of fluoride are primarily governed by pH and storage in bone. HF diffuses across cell membranes far more easily than fluoride ion. Because HF is a weak acid with a pKa of 3.4, more of the fluoride is in the form of HF when pH is lower. Consequently, pH—and factors that affect it—play an important role in the absorption, distribution, and excretion of fluoride. Fluoride is readily incorporated into calcified tissues, such as bone and teeth, substituting for hydroxyls in hydroxyapatite crystals. Fluoride exchanges between body fluids and bone, both at the surface layer of bone (a short-term process) and in areas undergoing bone remodeling (a longer-term process). Most of the fluoride in the body, about 99%, is contained in bone.
Fluoride is well absorbed in the alimentary tract, typically 70% to 90%. For sodium fluoride and other very soluble forms, nearly 100% is absorbed. Fluoride absorption is reduced by increased stomach pH and increased concentrations of calcium, magnesium, and aluminum. At high concentrations, those metals form relatively insoluble fluoride salts. A recent study comparing hard and soft water found little difference in fluoride bioavailability in healthy young volunteers (Maguire et al. 2004). Fluoride
can increase the uptake of aluminum into bone (Ahn et al. 1995) and brain (Varner et al. 1998).
Fluoride concentrations in plasma, extracellular fluid, and intracellular fluid are in approximate equilibrium. The concentrations in the water of most tissues are thought to be 40% to 90% of plasma concentrations, but there are several important exceptions. Tissue fluid/plasma (T/P) ratios exceed one for the kidney because of high concentrations in the renal tubules. T/P ratios can exceed one in tissues with calcium deposits, such as the placenta near the end of pregnancy. The pineal gland, a calcifying organ that lies near the center of the brain but outside the blood-brain barrier, has been found to accumulate fluoride (Luke 2001). Fluoride concentrations in adipose tissue and brain are generally thought to be about 20% of plasma or less (Whitford 1996). The blood-brain barrier is thought to reduce fluoride transfer, at least in short-term experiments (Whitford 1996). It is possible that brain T/P ratios are higher for exposure before development of the blood-brain barrier.
Most tissue measurements are based on short-term exposures of healthy adult animals. Similar T/P ratios have been found for liver and kidney in some chronic animal experiments (Dunipace et al. 1995), but not all organs have been examined. The literature contains some unexplained exceptions to these T/P generalizations (Mullenix et al. 1995; Inkielewicz and Krechniak 2003). Mullenix et al. (1995) reported atypically high, dose-dependent T/P ratios for the rat brain: more than 20 for control animals and about 3 for animals exposed to fluoride at 125 mg/L in drinking water for 20 weeks. Because these T/P ratios for brain are much higher than earlier results, Whitford (1996) speculated that the results of Mullenix et al. were due to analytical error. Additional measurements of fluoride tissue concentrations after chronic dosing are needed.
Fluoride is cleared from plasma through two primary mechanisms: uptake by bone and excretion in urine. Plasma clearance by the two routes is approximately equal in healthy adult humans. (Plasma clearance is the volume of plasma from which fluoride is removed per unit time. The rate of removal equals the clearance times the plasma fluoride concentration. Clearances are additive.) The relative clearance by bone is larger in young animals and children because of their growing skeletal systems. “In contrast to the compact nature of mature bone, the crystallites of developing bone are small in size, large in number and heavily hydrated. Thus, they afford a relatively enormous surface area for reactions involving fluoride” (Whitford 1996, p. 94). Experimental work in growing dogs demonstrates that extrarenal clearance, almost entirely uptake by bone, is inversely related to age. Renal clearance depends on pH and glomerular filtration rate. At low pH, more HF is formed, promoting reabsorption. Excretion of previously absorbed fluoride from the body is almost entirely via urine. Fluoride not absorbed
by the gut is found in feces. High concentrations of calcium in contents of the gastrointestinal tract can cause net excretion of fluoride.
Fluoride is rapidly absorbed from the gastrointestinal tract, with a half-life of about 30 minutes. After a single dose, plasma concentrations rise to a peak and then fall as the fluoride is cleared by the renal system and bone, decreasing back to (short-term) baseline with a half-life of several hours. Fluoride concentrations in plasma are not homeostatically controlled (Whitford 1996). Chronic dosing leads to accumulation in bone and plasma (although it might not always be detectable in plasma.) Subsequent decreases in exposure cause fluoride to move back out of bone into body fluids, becoming subject to the same kinetics as newly absorbed fluoride. A study of Swiss aluminum workers found that fluoride bone concentrations decreased by 50% after 20 years. The average bone ash concentration in the workers was about 6,400 mg/kg at the end of exposure, estimated via regression (Baud et al. 1978). The bone concentration found in these workers is similar to that found in long-term consumers of drinking water containing fluoride in the range of 2-4 mg/L (discussed later in this chapter). Twenty years might not represent a true half-life. Recent pharmacokinetic models (see below) are nonlinear, suggesting that elimination rates might be concentration dependent.
PHARMACOKINETIC MODELS
Pharmacokinetic models can be useful for integrating research results and making predictions. Two important fluoride models have been published since the 1993 NRC review. Turner et al. (1993) modeled bone concentrations in healthy adult humans. They assumed a nonlinear function relating the concentrations of fluoride in newly formed bone to plasma/ extracellular fluids. The relationship is close to linear until bone ash concentrations reach about 10,000 mg/kg; above that concentration the curve levels off. (Based on the chemical structure of fluorapatite, Ca10(PO4)6F2, the theoretical limit on bone fluoride concentration is 37,700 mg/kg.) The model was relatively successful at predicting fluoride bone concentrations due to chronic exposure compared with experimental data—for example, the human bone measurements of Zipkin et al. (1958). Bone fluoride concentrations were predicted to increase approximately linearly as a function of water concentration, at least up to 4 mg/L. The most sophisticated model to date (Rao et al. 1995) extended this work with a physiologically based pharmacokinetic (PBPK) model. Among other features, it models change in body weight, plasma clearance, and bone uptake as a function of sex and age, allowing predictions for lifetime exposures. It can model both rats and humans, making it useful for comparing these species. Predicted bone concentrations were comparable with data from several studies of humans,
including the study by Zipkin et al. (1958), and two rat carcinogenicity studies (Maurer et al. 1990; Bucher et al. 1991). Both models predicted increasing fluoride concentrations in bone with length of chronic exposure. None of these studies presented results for plasma.
Both models also performed well in predicting bone concentrations of fluoride resulting from osteoporosis treatment, involving about 25 mg of fluoride per day for up to 6 years. This suggests that the models can adequately predict the results of both long-term lower exposures (drinking water) and shorter-term, higher exposures (treatment regimes) by changing exposure assumptions.
The PBPK model of Rao et al. (1995) could be used in several ways, including (1) predicting bone concentrations in people after lifetime exposures to assumed water concentrations or other exposure scenarios, and (2) comparing plasma and bone fluoride concentrations in rats and humans with the same exposure. The Rao model is quite complicated and relies on several numerical functions not provided in the paper. The Turner model is more limited in scope, unable to compare species or take sex- and age-related effects into account, but it is much simpler. Not enough detail on either model was available to replicate them nor was the committee able to obtain operational versions of the models.
FLUORIDE CONCENTRATIONS IN HUMAN BONE VERSUS WATER CONCENTRATION
Remarkably few data are available for studying the association between fluoride in human bone and low-dose chronic exposure via drinking water. Although there are a number of cross-sectional studies comparing bone concentrations with water concentrations, very few contain estimates of length of exposure. Most studies are autopsies, as bone samples can be difficult to obtain from healthy living subjects. Among studies examining exposure to fluoride at 4 mg/L, Zipkin et al. (1958) provided the only data set that included exposure durations. The results of that study were also modeled by Turner et al. (1993) and Rao et al. (1995). Sixty-three of the 69 subjects, aged 26 to 90, died suddenly, primarily due to trauma, cardiovascular disease, and cerebrovascular causes; three had renal disease. The authors recorded concentrations of fluoride in drinking water and bone as well as sex, age, and years of residence. Compared with today, many other sources of fluoride exposure were uncommon or did not exist. The average residence time for the whole study was 31 years, 34 years for the 2.6-mg/L group and 21 years for the 4-mg/L group. Exposure took place for most people as adults. No estimates of water consumption are provided: water concentration serves as an ecologic measure of exposure.
Table 3-2 summarizes data on fluoride content of the iliac crest, the
TABLE 3-2 Fluoride in Bone Due to Chronic Water Exposurea
bone modeled by Turner et al. and Rao et al. Zipkin et al. concluded that average bone fluoride concentrations were linearly related to water concentration. (As discussed in Appendix C, this analysis is fully ecologic). The committee regressed individual-level bone concentrations versus water concentrations (a group measure of exposure) and individual-level covari-ates such as age. (This analysis is partially ecologic.) Figure 3-1 plots bone versus water concentrations and the result of simple regression with no covariates. (Note the apparent heteroscedasticity.) The model was improved
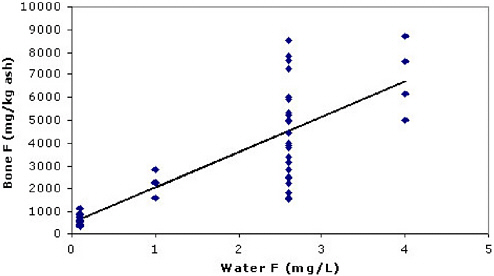
FIGURE 3-1 Illiac crest data from Zipkin et al. (1958). Crude regression results: y = 517 + 1,549x; (r2 = 0.66); slope = 1,549 (95% confidence interval [CI] = 1,227, 1,872).
by including residence years and sex; age had little additional impact and was omitted in the final model (Table 3-3).
Several cross-sectional studies have found an association between fluoride bone concentrations and age (Jackson and Weidmann 1958; Kuo and Stamm 1974; Parkins et al. 1974; Charen et al. 1979; Alhava et al. 1980; Eble et al. 1992; Richards et al. 1994; Torra et al. 1998). Jackson and Weidmann (1958) were unusual in finding a leveling off at an older age. But most studies did not have information on length of exposure, a variable often correlated with age (R = 0.41 in the Zipkin data set). Because of the potential for rapid fluoride uptake by bones during childhood, the committee modeled exposure before puberty with an indicator variable, but this added little to the model. Very few data are available on bone fluoride concentrations in children. Most studies do not distinguish between trabecular and cortical bone, although the former have higher fluoride concentrations (Eble et al. 1992).
The model in Table 3-3 indicates that fluoride bone concentrations increased with fluoride water concentrations and residence time; females tended to have higher concentrations than males. These results need to be interpreted with caution. Some subjects had renal disease, which can sometimes increase fluoride concentrations (see discussion below), potentially reducing the generalizability of the results to a healthier population. The committee’s analysis is partially ecologic (Appendix C). However, the Turner and Rao pharmacokinetic models also predict that fluoride bone concentrations increase with water concentration and duration of chronic exposure.
What bone fluoride concentration occurs after 70 years of exposure to water at 4 mg/L? The multiple regression model predicts about 8,100 mg/kg ash for females, within the range of the data set used to construct the model but near its maximum. Few people studied by Zipkin et al. were exposed for 70 years and only four were exposed at 4 mg/L. Fluoride is taken up by bone more rapidly during growth than in adulthood. This phenomenon, not addressed by the regression model, could cause the model to underpredict. Only the model of Rao et al. was constructed to examine lifetime exposure. Assuming 70 years of exposure at 4 mg/L in water, Rao et al. predicted fluoride concentrations of 10,000 to 12,000 mg/kg in bone ash for females. Even
TABLE 3-3 Multiple Regression Results for Zipkin Data
|
Coefficient |
95% CI |
P value |
Intercept |
−556 mg/kg |
(−1,512, 401) |
0.25 |
Water fluoride |
1,527 |
(1,224, 1,831) |
2.7 × 10−13 |
Residence, years |
26.5 mg/kg/year |
(7.48, 45.5) |
0.007 |
Sex (M = 0) |
663 mg/kg |
(−148, 1,475) |
0.11 |
higher values would be predicted if other sources of fluoride exposure were included. This prediction lies beyond the range of the human data used to check the model, but it represents the current best estimate. In making this prediction, the authors appear to have assumed consumption of 1 L of water per day up to age 10 and 2 L/day thereafter. Higher water consumption rates (e.g., 5 L/day) would further increase bone concentrations of fluoride but by less than fivefold because of the nonlinear kinetics.
Unfortunately, Rao et al. did not publish predictions for 2 mg/L. The regression model of Table 3-3 predicts about 5,000 mg/kg ash for females after 70 years of exposure. This value exceeds the mean value (4,500 mg/kg) observed at 2.6 mg/L in the Zipkin study, primarily because of the assumed longer time of residence. As this estimate is based on regression modeling of the Zipkin data, it may underestimate predictions based on pharmacokinetic modeling or additional sources of exposure. The committee located only a few other studies that measured bone fluoride at similar water concentrations. A British study found bone concentrations of about 5,700 mg/kg ash in people chronically exposed to water with fluoride at 1.9 mg/L; these people are also thought to be exposed to fluoride in tea (Jackson and Weidmann 1958; see Turner et al 1993 for unit conversions). In an area of rural Finland with fluoride in drinking water exceeding 1.5 mg/L, the average bone concentrations from 57 autopsies were 3,490 mg/kg ash in females and 2,830 mg/kg ash in males (Arnala et al. 1985). Most had lived their whole lives in the same place, most were over 50, and 7 had impaired renal function. For 16, fluoride concentrations were measured in the water sources (2.6 ± 1.4 mg/L); bone concentrations were 4,910 ± 2,250 mg/kg ash. In a later study of the same area of Finland, the mean bone concentration in 18 hip fracture patients was 3,720 ± 2,390 mg/kg, assumed to be ash (Arnala et al. 1986). The mean age was 79, 14 were female, 3 had diabetes, and 1 had elevated serum creatinine; residence time was not specified. For people exposed to fluoride at 2 mg/L in drinking water for a lifetime, the committee concludes that average bone concentration can be expected to be in the range of 4,000 to 5,000 mg/kg ash. Considerable variation around the average is expected.
FLUORIDE CONCENTRATIONS IN BONES AFTER CLINICAL STUDIES
A number of clinical studies measured bone fluoride concentrations after therapeutic treatment (van Kesteren et al. 1982; Boivin et al. 1988; Bayley et al. 1990; Gutteridge et al. 1990; Orcel et al. 1990; Boivin et al. 1993; Søgaard et al. 1994; Lundy et al. 1995). Figure 3-2 summarizes these data, plotting fluoride concentrations in bone ash after treatment versus total exposure from the studies. The weighted least squares (WLS) regression
line weighted points according to the number of participants in each trial (see Appendix C). Note that the two points farthest above the regression line (Bayley et al. 1990; Lundy et al. 1995) were from studies carried out in Toronto and Minnesota, presumably fluoridated areas; most (possibly all) of the other studies were conducted in European countries that do not fluoridate water. The two points farthest below the line delivered fluoride in a form designed to reduce bioavailability (Boivin et al. 1988, Turner et al. 1993). This analysis is ecologic, plotting average bone concentrations versus total exposure. However, analysis of individual-level data in two studies (van Kesteren et al. 1982; Gutteridge et al. 1990) provides similar results.
Because the pharmacokinetics of fluoride are nonlinear, we would not necessarily expect people with the same cumulative exposure to have the same bone fluoride concentrations. Indeed, the model may overpredict bone concentrations for long-term exposure to lower fluoride concentrations via water. Figure 3-2 also shows the average bone ash concentrations measured by Zipkin et al. for fluoride at 4 mg/L plotted against estimated total exposure. The latter was estimated assuming consumption of 1.51 L of water per day (Turner et al. 1993) and 21 years of exposure to fluoride in the 4-mg/L area. (The Zipkin study reported residence time and water concentrations but not water consumption.) While not completely out of range, the bone concentration is lower than expected based on the regression for the clinical data. Analysis of Turner’s pharmacokinetic model (Turner et al. 1993) suggests that short-term (months to years), high-dose exposures
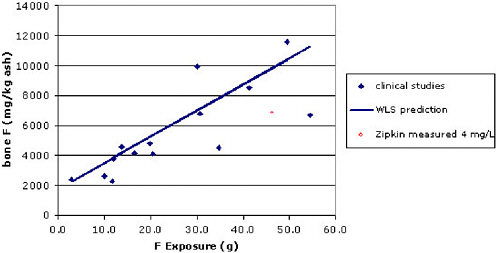
FIGURE 3-2 Bone fluoride concentrations versus total exposure in clinical trials. For comparison, the average bone concentration found by Zipkin et al. (1958) among subjects drinking water with fluoride at 4 mg/L is provided.
may produce higher bone fluoride concentrations than long-term (decades), low-dose exposures. More time means more bone resorption, allowing a greater fraction of the total fluoride dose to be excreted. Additional research on this topic would be useful.
More detailed information on fluoride’s effects on bone cells and bone formation is presented in Chapter 5.
COMPARATIVE PHARMACOKINETICS OF RATS AND HUMANS
Among animal species, fluoride toxicology has been studied most extensively in rats. When extrapolating from rats to humans, it is useful to consider their relative pharmacokinetics. There are at least two ways to do this. Bone, tissue, or plasma concentrations may provide an appropriate biomarker of internal exposure for some effects. Alternatively, one can compare plasma, tissue, and bone concentrations in rats and humans given the same dose.
Our knowledge of the comparative pharmacokinetics of fluoride is primarily limited to short-term studies of a small number of mammals. Using estimates of plasma, renal, and extrarenal fluoride clearances scaled to body weight, Whitford et al. (1991) concluded that dogs were the best pharmacokinetic model for humans, based on studies of healthy young adults. In contrast, renal clearance in rats (age 12 weeks) was more than three times larger than in humans; rat extrarenal clearance was about twice as large (Whitford et al. 1991). Unlike in humans, rat bones do not undergo Haversian remodeling (remodeling along channels within the bone). Fluoride uptake by the bones of adult rats should be minimal (Turner et al. 1995).
Comparisons between species—and within species for different experiments—are complicated by several factors. With chronic exposure, fluoride bone concentrations tend to increase over time. The amount of calcium in the diet affects the amount of fluoride absorbed. The dose of fluoride can depend on the concentration of fluoride in water, water consumption, and the amount of fluoride in the diet. If fluoride concentration is kept constant in water, dose can vary as the animal ages. Species age at different rates, and age affects pharmacokinetics, especially bone development and kidney function.
Evidence suggests that rats require higher chronic exposure than humans to achieve the same plasma and bone fluoride concentrations. It has been suggested that rats might require water concentrations about five times larger than humans to reach the same plasma concentration (Dunipace et al. 1995). For bone, Turner et al. (1992) estimated that “humans incorporate fluoride ~18 times more readily than rats when the rats are on a normal calcium diet.” This comparison was also based on water concentrations. In Appendix D, this issue is briefly reviewed. The factor for plasma is uncertain, in
part because it could change with age or duration of dose. It might be more appropriate to compare exposures than water concentration. Bone comparisons are also uncertain but appear to support a rat-to-human conversion factor for older rats and humans of at least an order of magnitude.
ORGANOFLUORINE COMPOUNDS
Two types of fluorine are found in human plasma: inorganic and organic. Up to now, this chapter has discussed the inorganic form. Remarkably, the amount of organic fluoride in serum is generally greater than the amount of inorganic fluoride (Whitford 1996). Interest in organofluorine compounds has grown tremendously in the last decade. Two compounds (and their salts) dominate recent biological research: perfluorooctanesulfonate (PFOS; C8F17SO3−) and perfluorooctanoate (PFOA; C7F15COO−). Both are straight-chain compounds with fluorine substituted for aliphatic hydrogens. These compounds are biologically stable with long half-lives, on the order of years, in humans. Relatively little is known about the routes of human exposure. A recent study of American Red Cross adult blood donors found median serum concentrations of 35 µg/L of PFOS and 5 µg/L of PFOA (Olsen et al. 2003).
Defluorination of PFOA has not been detected in rat experiments (Vanden Heuvel et al. 1991; Kudo and Kawashima 2003). Given the stability of PFOA and PFOS, they do not appear to be important sources of inorganic fluoride, although more research is needed, particularly for PFOS. Degradation of other fluorocarbons might produce fluoride ion. Perfluorooctanesulfonyl fluoride (POSF, C8F17SO2F) is used as a starting material for manufacturing polymers and surfactants. Residual POSF in products “may degrade or metabolize, to an undeterminate degree” to PFOS (Olsen et al. 2004, p. 1600). Certain anesthetics release fluoride ion during use (see Chapter 2).
FACTORS MODIFYING PHARMACOKINETICS AND THEIR IMPLICATIONS FOR POTENTIALLY SUSCEPTIBLE POPULATIONS
Changes in chronic exposure to fluoride will tend to alter plasma and bone fluoride concentrations. A number of factors can modify the pharmacokinetics, providing another way to change fluoride tissue concentrations.
Fluoride clearance tends to increase with urinary pH. One proposed mechanism is decreased reabsorption in the renal tubule, easily crossed by HF and nearly impermeable to fluoride ion. Increasing urinary pH thus tends to decrease fluoride retention. As a result, fluoride retention might be affected by environments or conditions that chronically affect urinary pH,
including diet, drugs, altitude, and certain diseases (e.g., chronic obstructive pulmonary disease) (reviewed by Whitford 1996).
Because of their growing skeleton, infants and children clear relatively larger amounts of fluoride into bones than adults (Ekstrand et al. 1994; Whitford 1999). As discussed earlier, fluoride plasma and bone concentrations tend to increase with age. Although this trend is partly due to accumulation over time, decreased renal clearance and differences in bone resorption (preferential removal of cystallites with little or no fluoride in the elderly have been hypothesized to play a role.
Because the kidney is the major route of excretion, increased plasma and bone fluoride concentrations are not surprising in patients with kidney disease. Plasma fluoride concentrations are clearly elevated in patients with severely compromised kidney function, reduced glomerular filtration rates of around 20% of normal, as measured via creatinine clearance or serum creatinine concentrations (Hanhijärvi 1974, 1982; Parsons et al. 1975; Schiffl and Binswanger 1980; Waterhouse et al. 1980; Hanhijärvi and Penttilä 1981). Kuo and Stamm (1975) found no association. However, elevated serum concentrations were found in renal patients with normal serum creatinine (Hanhijärvi 1982).
Only a few studies have examined fluoride concentrations in bone in renal patients. Call et al. (1965) found doubled bone fluoride concentrations in five patients with chronic, severe kidney disease. Juncos and Donadio (1972) diagnosed systemic fluorosis (but did not measure bone fluoride concentrations) in two patients with reduced renal function and exposure to drinking water with fluoride at 1.7 and 2.6 mg/L. Four renal patients with severe skeletal changes or bone pain had elevated serum and bone fluoride concentrations; the bone concentrations ranged from about 5,500 to 11,000 mg/kg (Johnson et al. 1979). Fluoride bone concentrations more than doubled in four patients with severe, chronic pyelonephritis (Hefti and Marthaler 1981). Arnala et al. (1985) reported elevated bone concentrations (roughly 50%) in six people with “slightly impaired renal function” from a fluoridated area. Bone fluoride concentrations were significantly increased in dialysis patients compared with normal controls (Cohen-Solal et al. 2002). In rats with surgically induced renal deficiency (80% nephrectomy), glomerular filtration rate decreased by 68%. After 6 months of fluoride treatment, bone fluoride concentrations approximately doubled (Turner et al. 1996).
Hanhijärvi and Penttilä (1981) reported elevated serum fluoride in patients with cardiac failure. Fluoride concentrations were positively related to serum creatinine, although the concentrations of the latter did not indicate renal insufficiency. During cardiac failure, the body tries to maintain blood flow to the heart and brain.
Although some studies report no difference in plasma fluoride concen-
trations between men and women (e.g., Torra et al. 1998), others found greater rates of increase with age in females (Husdan et al. 1976; Hanhijärvi et al. 1981). Enhanced release of fluoride in postmenopausal women is one possible explanation. Similar to our regression results of the Zipkin data, some studies have found a tendency toward elevated bone fluoride concentrations in women (Arnala et al. 1985; Richards et al. 1994). A Finnish study reported that bone fluoride concentrations increased more rapidly with age in women than in men (Alhava et al. 1980). This variability might be due to several factors, including individual differences in water consumption and pharmacokinetics.
In sum, although the data are sparse, severe renal insufficiency appears to increase bone fluoride concentrations, perhaps as much as twofold. The elderly are at increased risk of high bone fluoride concentrations due to accumulation over time; although less clear, decreased renal function and gender may be important.
FINDINGS
-
Bone fluoride concentrations increase with both magnitude and length of exposure. Empirical data suggest substantial variations in bone fluoride concentrations at any given water concentration.
-
On the basis of pharmacokinetic modeling, the current best estimate for bone fluoride concentrations after 70 years of exposure to fluoride at 4 mg/L in water is 10,000 to 12,000 mg/kg in bone ash. Higher values would be predicted for people consuming large amounts of water (>2 L/day) or for those with additional sources of exposure. Less information was available for estimating bone concentrations from lifetime exposure to fluoride in water at 2 mg/L. The committee estimates average bone concentrations of 4,000 to 5,000 mg/kg ash.
-
Groups likely to have increased bone fluoride concentrations include the elderly and people with severe renal insufficiency.
-
Pharmacokinetics should be taken into account when comparing effects of fluoride in different species. Limited evidence suggests that rats require higher chronic exposures than humans to achieve the same plasma and bone concentrations.
RESEARCH RECOMMENDATIONS
-
Additional research is needed on fluoride concentrations in human bone as a function of magnitude and duration of exposure, age, gender, and health status. Such studies would be greatly aided by noninvasive means of measuring bone fluoride. As discussed in other chapters of this report, some soft tissue effects may be associated with fluoride exposure. Most measure-
-
ments of fluoride in soft tissues are based on short-term exposures and some atypically high values have been reported. Thus, more studies are needed on fluoride concentrations in soft tissues (e.g., brain, thyroid, kidney) following chronic exposure.
-
Research is needed on fluoride plasma and bone concentrations in people with small to moderate changes in renal function as well as patients with serious renal deficiency. Other potentially sensitive populations should be evaluated, including the elderly, postmenopausal women, and people with altered acid-base balance.
-
Improved and readily available pharmacokinetic models should be developed.
-
Additional studies comparing pharmacokinetics across species are needed.
-
More work is needed on the potential for release of fluoride by the metabolism of organofluorines.