6
Why Water? Toward More Exotic Habitats
A liquid phase facilitates chemical reactions, something that has been known empirically for centuries. As a solvent, a liquid allows dissolved reactants to encounter each other at rates that are higher than the rates of encounter between species in a solid. At the same time, a solvent provides an environment that has some degree of constancy relative to the spectrum of possible environments. In water, for example, the temperature must lie between 273 and 373 K at typical pressures, and the dielectric constant and other physical parameters that influence reaction rates are also within specific ranges. Chemical reactions can take place in the gas and solid phases as well, of course. But each of these phases has disadvantages relative to the liquid phase.
Results obtained from both the emerging field of synthetic biology and the analysis of origins are beginning to be effective in constraining the biomolecular form that life might have taken if it arose in water. Earlier chapters of this report discuss important chemical properties of water and their relevance to life. In this chapter the committee examines alternative perspectives suggesting that water might not be essential for life. Other than preferring a repeating charge in a genetic biopolymer able to support Darwinian evolution subject to the constraints of general chemistry, only a few arguments indicating that water is uniquely suited as a biosolvent survive scrutiny.
6.1
IS WATER UNIQUELY SUITED FOR LIFE?
Many specific properties of water are cited to make the case that water is an ideal biosolvent uniquely suited to support life: Frozen water floats. Water is an excellent solvent for salts. Water is liquid over a broad range of temperature. Indeed, the concept of a habitable zone, a region around a star where life is presumed to be possible, largely posits a region in which a planet’s surface or subsurface might support liquid water. One such planet in a habitable zone could export life to other suitable environments via meteoroids.
The committee found that many current views about water in relation to life are geocentric. For example, floating water ice can indeed insulate a body of liquid water below and thus keep it from freezing. However, several types of water ice exist. Ice 1, which is the most stable form of ice at 273 K at standard pressure, is indeed less dense than liquid water at those conditions. In contrast, ice 2, or any of the other form of ice, is denser than water. Those forms of ice sink. Researchers view ice 1 as especially worthy of discussion, because they live on an Earth-sized body. If researchers lived on a substantially more massive body, they might consider ice 1 to be less important than the forms of ice that sink.
Although surface ice does insulate a body of liquid water below from loss of heat, it also has a higher albedo than water liquid. When water ice floats, it reflects more light from the Sun, which leads to more cooling, more ice on the surface, a resultant higher albedo, and still more cooling. Floating water ice amplifies a glaciation event, leading to more glaciation. Indeed, it appears to have done just this many times on Earth, including within the past few million years. Thus, the fact that water ice floats causes water to amplify, not damp, perturbations in energy flux coming to a planet. If a useful property of a bulk solvent is that it supports a stable environment conducive to life, then the fact that water ice floats might be viewed as a disadvantage.
Another example is that a focus on terran sea-level atmospheric pressure can influence researchers’ views of the range of temperatures at which water is a liquid. On the surface of Mars liquid water cannot exist, except possibly transiently, because the pressure is too low, and water ice sublimes directly to water vapor without going through an intermediate liquid phase. Further, over most ranges of pressure, formamide has a larger liquid temperature range (255 to 480 K) than water and is also an excellent solvent for polar materials.
The view of water as an ideal biosolvent is geocentric in other ways. Although the temperature range over which water is a liquid is large on the Kelvin scale, it is important not to be tied to a linear view of the Celsius scale. The temperature range from 1 to 2 K is much more significant than from 273 to 274 K, both in fractional terms and in terms of how physical processes occur over that range.Although ammonia is liquid at lower temperatures than water, the temperature range over which ammonia is liquid for relevant planetary surface pressures is greater than for water.
Considered in a cosmological context, water is a liquid over a temperature range present in only a very small number of objects in the universe. Assuming that a fluid is necessary for life, and accepting the premise that water has properties that make it well suited to support life in the cosmos, what do water’s freezing and boiling points imply about the likelihood of its fluidity over a significant range of the space in the galaxy, or even around a star? This question has been examined recently by Ward and Brownlee,1 who concluded that complex life (i.e., animal life) is distributed sparsely, perhaps extremely sparsely,in the cosmos precisely because it requires water as a liquid, and that liquid water is sparsely distributed in the cosmos. However, Kasting has raised questions about this conclusion.2
Despite the apparent match between terran metabolism and water, water does not seem to be unquestionably preferable as a biosolvent. For example, although much of terran metabolism exploits C=O molecules, the electrophilicity of carbon doubly bonded to nitrogen (C=N) is equally satisfactory for supporting carbon-carbon bond-forming reactions. Yet, with the exception of aromatic heterocycles such as the purines, C=N is hardly used in terran biochemistry, because most other compounds containing C=N units spontaneously hydrolyze in water to generate the corresponding C=O compound and the corresponding N-containing by-product. But if a solvent other than water were the matrix for life, and if that solvent tolerated C=N units, would life not have been able to evolve in that solvent to exploit C=N units just as effectively as terran life today exploits C=O units?
The ability of water to form strong hydrogen bonds disrupts the hydrogen bonding useful for supramolecular structures. Water does not support protein folding, because it disrupts hydrogen bonds that stabilize the fold. Indeed, an examination of the chemical literature for examples of work on self-organizing molecules indicates that chemists consciously trying to achieve this outcome rarely use water, precisely because it disrupts noncovalent directional bonding such as hydrogen bonding. The situation is similar for genetic molecules. The DNA double helix is joined by hydrogen bonds that in water are only barely stable because water offers opportunities for a single strand of DNA to form hydrogen bonds, not with its complementary strand, but with water itself. But DNA, because it has a repeating charge in its backbone, is not expected to work well in nonwater solvents. This implies that a genetic molecule in nonwater solvents would not be DNA, or not be a polyelectrolyte. Conversely, if one accepts the notion of repeating charges as universal in genetic molecules, then one might have to conclude that a somewhat polar solvent (like water) is necessary for life.
Nevertheless, certain features of terran metabolism might benefit from a biosolvent whose properties differ from water’s. For example, the instability of C=N in water constrains the structure of metabolites in water. The compound HN=C=NH, an analog of O=C=O (carbon dioxide), immediately hydrolyzes in water to give urea (H2NCO-NH2), whose thermodynamic instability with respect to hydrolysis in water yields carbon dioxide and ammonia. Thus, water as a solvent requires that the dominant form of carbon at the +4 oxidation state be carbon dioxide.
Water and carbon dioxide, however, are a problematic pair, at least at terran sea-level atmospheric pressure. The carbon of carbon dioxide is a good electrophilic center. But carbon dioxide itself is poorly soluble in water (0.88 v/v at 293 K and 1 atm) and dissolves at pH 7 primarily in the form of the bicarbonate anion. Bicarbonate, however, has its electrophilic center shielded by the anionic carboxylate group and so is intrinsically unreactive as an electrophile. Thus, the metabolism of carbon dioxide is caught in a conundrum. The reactive form is insoluble; the soluble form is unreactive.
Terran metabolism has worked hard to manage this conundrum. The reactivity of the vitamin biotin was discussed nearly three decades ago in this context.3 Biotin is metabolically costly, however, and cannot be used to manage carbon dioxide and its problematic reactivity in large amounts. Although the enzyme ribulose bisphosphate carboxylase attempts to manage the problem without biotin, the problematic reactivity of carbon dioxide competes with the problematic reactivity of dioxygen. Even in highly advanced plants, a sizable fraction of the substrate intended to capture carbon dioxide is destroyed through reaction with dioxygen.4 Terran life, in nearly a billion years, has not found a compelling solution to this problem, which may be universal. Indeed, if we do encounter nonterran carbon-based life that lives in water, it will be interesting to see how it has come to manage the unfortunate properties of carbon dioxide.
6.2
IF NOT WATER, THEN WHAT SOLVENT?
Nature presents a large number of atomic and small molecular species that might be discussed as biosolvents. Table 6.1 lists some of these, together with their freezing and normal (i.e., at 1 atmosphere) boiling points. It is important to note another contribution of pressure to physical properties. The physical properties of the substances listed the Table 6.1 are described by a phase diagram that relates the state of a material (solid of various types, liquid, or gas) to temperature and pressure. Above a critical point in the phase diagram, the substance is a supercritical fluid, neither liquid nor gas. Table 6.2 shows the critical temperatures and pressures for some substances common in the solar system.
The properties of supercritical fluids are generally different from those of regular fluids. For example, supercritical water is relatively nonpolar and acidic. Further, the properties of a supercritical fluid, such as its density and viscosity, change with changing pressure and temperature, dramatically as the critical point is approached. Thus, carbon dioxide is not listed in Table 6.1 because it has no liquid phase at terran atmospheric pressure. Carbon dioxide has a critical temperature of 304.2 K and pressure of 73.8 atm, however. It is therefore a supercritical fluid above that pressure, and may even exist as a potential biosolvent for rocky planets having the approximate mass of Earth (or Venus).
TABLE 6.1 Freezing and Boiling Points (at 1 atm) of Some Solvents
Solvent |
Freezing Point (K) |
Boiling Point (K) |
Ammonia |
195 |
240 |
Dihydrogen |
14 |
20 |
Dinitrogen |
63 |
77 |
Ethane |
101 |
184 |
Formamide |
273 |
495 |
Helium |
— |
4 |
Hydrazine |
275 |
387 |
Hydrogen cyanide |
260 |
299 |
Hydrogen fluoride |
190 |
293 |
Hydrogen sulfide |
192 |
213 |
Methane |
91 |
112 |
Neon |
25 |
27 |
Sulfuric acid |
283 |
563 |
Water |
273 |
373 |
TABLE 6.2 Critical Temperature and Pressure for Selected Substances
Liquid |
Critical Temperature (K) |
Critical Pressure (atm) |
Hydrogen |
33.3 |
12.8 |
Neon |
44.4 |
26.3 |
Nitrogen |
126 |
33.5 |
Argon |
151 |
48.5 |
Methane |
191 |
45.8 |
Ethane |
305 |
48.2 |
Carbon dioxide |
305 |
72.9 |
Ammonia |
406 |
112 |
Water |
647 |
218 |
It is useful to divide the solvents into three groups: polar solvents that are not water, nonpolar solvents, and cryosolvents.
6.2.1
Polar Solvents That Are Not Water
6.2.1.1
Ammonia
Ammonia is analogous to water in many of its properties. Ammonia, like water, dissolves many organic compounds, including many polyelectrolytes. Preparative organic reactions are often done in ammonia in the laboratory. Ammonia, like water, is liquid over a wide range of temperatures (195 to 240 K at 1 atm). The liquid range is even broader at higher pressure. For example, at 60 atm ammonia is liquid from 196 to 371 K. Further, liquid ammonia may be abundant in the solar system. A large amount of the inventory of liquid ammonia in the solar system exists, for example, in clouds in the jovian atmosphere. However, as noted earlier by the committee, some view clouds as unlikely places to harbor life. However, if clouds are not transient and broken (as on Earth) but are rather more continuous (as on Venus), this view may need modification.
As compared with water, ammonia’s increased ability to dissolve hydrophobic organic molecules suggests an increased difficulty in using the hydrophobic effect to generate compartmentalization in ammonia, relative to water. This in turn implies that the liposome, a compartment that works in water, generally will not work in liquid ammonia. Hydrophobic phase separation is possible in ammonia, however, albeit at lower temperatures. For example, Brunner reported that liquid ammonia and hydrocarbons form two phases, where the hydrocarbon chain contains from 1 to 36 CH2 units.5 Different hydrocarbons become miscible with ammonia at different temperatures and pressures. Thus, formation of ammonia-phobic and ammonia-philic phases, analogous to the hydrophobic and hydrophilic phases in water, useful for isolation would be conceivable in liquid ammonia at temperatures well below its boiling point at standard pressures.
The greater basicity of liquid ammonia must also be considered. The species that serve as acid and base in pure water are H3O+ and HO−. In ammonia, NH4+ and NH2− are the acid and base, respectively. H3O+, with a pKa of −1.7, is about 11 orders of magnitude stronger (in water) as an acid than NH4+, with a pKa of 9.2 (in water). Likewise, NH2− is about 15 orders of magnitude stronger as a base than HO−.
The increased strength of the dominant base in ammonia,as well as the corresponding enhanced aggressivity of ammonia as a nucleophile, implies that ammonia would not support the metabolic chemistry found in terran life. Terran life exploits compounds containing the C=O carbonyl unit. In ammonia, carbonyl compounds are (at the very least) converted to compounds containing the corresponding C=N unit. Nevertheless, hypothetical reactions that exploit a C=N unit in ammonia can be proposed in analogy to the metabolic biochemistry that exploits the C=O unit in terran metabolism in water (Figure 6.1).6 Given this adjustment, metabolism in liquid ammonia is easily conceivable.
Most interestingly, ammonia is a potent antifreeze for water. Recently recovered data from Titan suggest that that moon is periodically being resurfaced by a liquid having a viscosity comparable to that of a water-ammonia eutectic, which is liquid even in an environment that experiences methane rain. Water-ammonia eutectics, which
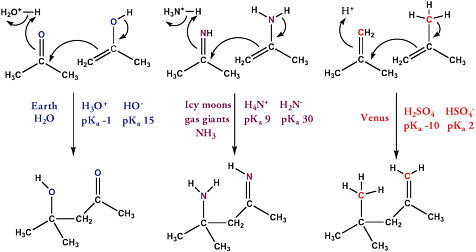
FIGURE 6.1 Different functional groups, but analogous mechanisms, could be used to form new C—C bonds in different solvents. In water, the C=O unit would provide the necessary reactivity. In ammonia, the C=N unit would provide the necessary reactivity. In sulfuric acid, the C=C unit is sufficient to provide the necessary reactivity.
are liquid even at the temperature of Titan, are a potential biosolvent. They are abundant in the cosmos, they have a wide temperature range of liquidity, and they are not bad as solvents.
6.2.1.2
Sulfuric Acid as a Possible Solvent
Ammonia is not the only polar solvent that might serve as an alternative to water. For example, sulfuric acid is a reasonably good solvent that supports chemical reactivity.7 Sulfuric acid is known to exist above Venus,8 where three cloud layers at 40 to 70 km are composed mostly of aerosols of sulfuric acid, about 80 percent in the upper layer and 98 percent in the lower layer. The temperature (about 310 K at about 50 km altitude, at about 1.5 atm) is consistent with stable carbon-carbon covalent bonds. Many authors have discussed the possibility of early life on Venus in its acidic environment.9-13 The surface temperature of Venus is approximately 740 K. Sagan and Morowitz even considered organisms that float above the hot surface using hydrogen “float bladders”14 analogous to those found in terran aquatic organisms, although others have raised questions about how to explain the prior evolution of a float bladder that would let an organism control its altitude.a Schulze-Makuch et al. argued for sample return from the venusian atmosphere to address the possibility of life there.15
Hypotheses about metabolism are abundant for the hypothetical life in acidic aerosols. In strong acid, the C=C bond is reactive as a base and can support a metabolism as an analog of the C=O unit. This type of chemical reactivity is exemplified in some terran biochemistry. For example, acid-based reactions of the C=C unit have been used by plants as they synthesize fragrant molecules.16
Nor are sources of energy in short supply in the venusian atmosphere. For example, a venusian metabolism might exploit the relatively high flux of ultraviolet radiation in the venusian clouds.17
6.2.1.3
Formamide as a Possible Solvent
Formamide (HCONH2) is another potential biosolvent. Formamide is formed by the reaction of hydrogen cyanide with water; both are abundant in the cosmos. Like water, formamide has a large dipole moment and is an excellent solvent for almost anything that dissolves in water, including polyelectrolytes. In particular, formamide is able to dissolve RNA, DNA, and proteins, as well as their precursors. Formamide is not reactive like water. Indeed, many species that are thermodynamically unstable in water with respect to hydrolysis, are stable in formamide.
Formamide is itself hydrolyzed by water, meaning that it persists only in a relatively dry environment, such as a desert. Desert environments recently proposed as being potential sites for the prebiotic synthesis of ribose18 may hold formamide as well. Since formamide boils at ~400 K, a mixture of formamide and water, if placed in the desert, would lose its water over time and end up as a pool of formamide. Within this pool, many syntheses are thermodynamically favorable: polypeptides from amino acids, nucleosides from sugars and bases, nucleotides from nucleosides and inorganic phosphate, and RNA from nucleotides. Indeed, phosphate esters are also spontaneously synthesized. This includes ATP (from ADP and inorganic phosphate), nucleosides (from ribose borates and nucleobases), peptides (from amino acids), and others.19-21
6.2.2
Nonpolar Solvents
A variety of solvents have higher boiling points than that of water but do not have polar structures. The most accessible of these are the hydrocarbons, which come in a series from the smallest (methane) to higher homologs (ethane, propane, butane, and so on) and are abundant in the solar system. Methane, ethane, propane, butane, pentane, and hexane have boiling points of about 109, 184, 231, 273, 309, and 349 K, respectively, at standard terran pressure. Thus, at a mean surface temperature of 95 K, methane (which freezes at 90 K) would be liquid, implying that oceans of methane could cover the surface of Titan.
Many discussions of life on Titan have considered the possibility that water, normally frozen at the ambient temperature, might remain liquid following heating by impacts.22 Life in this aqueous environment would be subject to the same constraints and opportunities as life in water. Water droplets in hydrocarbon solvents are, in addition, convenient cellular compartments for evolution, as Tawfik and Griffiths have shown in the laboratory.23 An emulsion of water droplets in oil is obtainable by simple shaking. This could easily be a model for how life on Titan achieves the isolation necessary for Darwinian evolution, and it provides an interesting alternative for membranes, discussed in earlier chapters as a common feature of terran life.
Broad empirical experience shows that organic reactivity in hydrocarbon solvents is no less versatile than in water. Indeed, many terran enzymes are believed to catalyze reactions by having an active site that is not water-like. Further, with ethane as a solvent, a hypothetical form of life would be able to use hydrogen bonding more effectively; these bonds would have the strength appropriate for the low temperature. Further, hydrocarbons with polar groups can be hydrocarbon-phobic; acetonitrile and hexane, for example, form two phases. It is possible to conceive of liquid/liquid phase separation in bulk hydrocarbons that could achieve the isolation necessary for Darwinian evolution.
Because of its reactivity, water destroys hydrolytically unstable organic species. Thus, a hypothetical form of life in a Titan hydrocarbon ocean would be less subject to the hydrolytic deamination of its nucleobases, and would be able to guide reactivity more easily than life in water.
Thus the environment of Titan meets the absolute requirements for life. Titan is not at thermodynamic equilibrium. It has abundant carbon-containing molecules and heteroatoms and a fluid environment. Titan’s temperature is low enough to permit a wide range of bonding, covalent and noncovalent. Titan undoubtedly offers other resources believed to be useful for catalysis necessary for life, including metals and surfaces.
This makes inescapable the conclusion that if life is an intrinsic property of chemical reactivity, life should exist on Titan. Indeed, for life not to exist on Titan, we would have to argue that life is not an intrinsic property
of the reactivity of carbon-containing molecules under conditions where they are stable. Rather, we would have to conclude that either life is scarce in these conditions or that there is something special, and better, about the environment that Earth presents (including its water).
6.2.3
Cryosolvents
Many of the most important potential solvents found in the solar system exist only in their gaseous form on Earth. They become liquids at temperatures that are (regarded by humans as being) low. Hence, they are known as cryosolvents. Low temperatures are, however, prominent throughout the cosmos, as are species that are liquid there. Therefore, cryosolvents cannot be dismissed as potential biosolvents.
6.2.3.1
Dihydrogen
The most abundant compound in the solar system is dihydrogen, the principal component (86 percent) of the upper regions of the gas giants Jupiter, Saturn, Uranus, and Neptune. The other principal component of the outer regions of the giant planets is helium (14 percent). Throughout most of the volume of gas giants where dihydrogen is stable, it is a supercritical fluid. For the gas giants, two radii can be defined. The first is the radius of the region where dihydrogen becomes supercritical. The second is where the temperature rises to a point where organic molecules are no longer stable; for this discussion, 500 K is chosen as that point. If the second radius is smaller than the first, then the gas giant has a habitable zone for life in supercritical dihydrogen. If the second radius is larger than the first, however, then the planet has no habitable zone.
If such a zone exists on Jupiter, it is narrow. Where the temperature is 300 K (clearly suitable for organic molecules), the pressure (about 8 atm) is still subcritical. At about 200 km down, where the jovian pressure is supercritical, the temperature rises above 500 K, approaching the upper limit where carbon-carbon bonds are stable.24
For Saturn, Uranus, and Neptune, the habitable zone is broader relative to the planetary radius. On Saturn, the temperature is about 300 K when dihydrogen becomes supercritical. On Uranus and Neptune, the temperature when dihydrogen becomes supercritical is only 160 K, a temperature at which organic molecules are stable.
The atmospheres of these planets convect, of course. To survive on Jupiter, any hypothetical life based on molecules containing carbon-carbon covalent bonds would have to avoid being moved by convection to positions in the atmosphere where they are not stable. This is, of course, not impossible. Even on Earth, life in the oceans must avoid being moved by convection from its particular habitable zone. Sagan and Salpeter presented a detailed discussion of what might be necessary for a “floater” to remain stable in the jovian atmosphere.25
Bains has recently discussed dinitrogen as a possible biocryosolvent.26 His article also reviews the principal issues in using a cryosolvent. The most significant is the relative insolubility of substances in cryosolvents.
Thus, little is known about the behavior of organic molecules in supercritical dihydrogen as a solvent. In the 1950s and 1960s, various laboratories studied the solubility of organic molecules (e.g., naphthalene) in compressed gases, including dihydrogen and helium.27,28 None of the environments examined in the laboratory explored high pressures and temperatures, however.
6.2.3.2
Dinitrogen
Bains considered the possibility of organic species being dissolved in dinitrogen, including the possibility that silicon-based species might have greater solubility in dinitrogen than carbon-based species.29 However, the reactivity of silanes would make them unworkable as biopolymers on today’s Earth, because water reacts with many silanes.
This is not the case for dinitrogen as a biosolvent. Dinitrogen is abundant in the cosmos, like water. Its lower freezing and boiling points, however, make it a liquid over a wider range of the cosmos. For example, liquid dinitrogen may be a bulk solvent on Triton, the largest moon of Neptune.
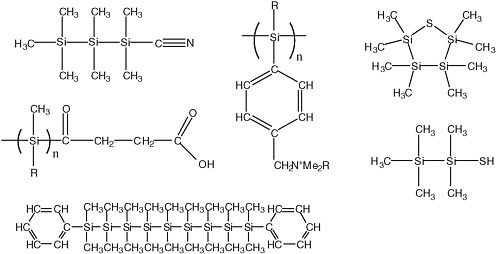
FIGURE 6.2 Structures of some synthetic polysilanes that have been described in the literature.
A range of silicon-containing compounds is known (see Figure 6.2). There is little question that polymeric diversity is possible using silicon, rather than carbon, as a scaffolding.
One difficulty with cryosolvents is the slow rate of chemical reactions. Here again, it is important not to be geocentric. Chemical reactions of the type known in water that occur between 273 and 373 K are indeed slow at 100 K. However, a wide range of chemical reactions that are too fast to be considered as part of terran metabolism—such as reactions involving the making and breaking of hydrogen bonds—become slower at cryotemperatures. At cryotemperatures, such reactions might be productively controlled by catalysts that could be under the control of genetic molecules in a hypothetical life form.
6.2.3.3
Other Supercritical Cryosolvents
As noted in Tables 6.1 and 6.2, many of the most abundant potential solvents in the solar system are supercritical in their most abundant environments.
Supercriticality in an environment does not, in itself, prohibit life. Some terran enzymes are known to be active in supercritical fluids.30-32 Subsequent reviews can be found in Aaltonen and Rantakyla,33 Kamat et al.,34 and Aaltonen.35 Although most of that work concerns supercritical carbon dioxide as the solvent, fluorinated hydrocarbons (HCF3) and simple alkanes (e.g., ethane, propane) have also been reported,36 providing a formal demonstration that terran-derived proteins can function in these media. Any enzyme adapted to the supercritical media would undoubtedly be different from those used in the studies cited.
Organic chemists have been attracted for a variety of reasons to supercritical media as an environment for performing reactions. These reasons include, especially for CO2 and H2O, the environmental friendliness of the medium. The fact that supercritical fluids can be removed without a residue is an advantage. Other advantages include the solubility of gases within supercritical mixtures, the high diffusion rates, and the variable and adjustable density, solvent power, and dielectric constant of the medium. Ordinary gases, such as O2 and H2, are miscible with
supercritical fluids, such as supercritical CO2. That property removes a limitation that is well known in standard liquids, including water, in which reactions are limited by the poor solubility of gases in the standard liquid. Table 6.1 shows some of the solvents studied.
Supercritical water is a key species in mineralogy, as well as in the commercial synthesis of crystalline inorganic species, including alpha-quartz and gemstones.37 It has rarely been used in organic chemistry, although there are examples of its use for the oxidation of methane, the degradation of biopolymers, and the dehydration of alkenes.38 Its high critical temperature (647 K) implies that many organic species are unstable in supercritical water. Nevertheless, some reactions are known.39
6.3
STILL MORE EXOTIC HABITATS
6.3.1
Life in the Gas Phase
In the gas phase, chemistry is limited to molecules that are sufficiently volatile under the ambient conditions to deliver an adequate amount of material to the gas phase at moderate temperatures. Raising the temperature does not necessarily solve the problem. Although more molecules enter their gas phases as the temperature is increased, organic molecules generally cease to be stable at temperatures in excess of 500 K.
It is conceivable that in the vacuum of interstellar space, life exists through molecules at high dilution reacting in the gas phase. Grains have been suggested as a way of collecting organic species, perhaps with an outer layer that protects the inner layer from irradiation. The possible disadvantage of this, of course, is the difficulty in holding together the components of the interstellar life form. One advantage of such a life form would be that it need not be encumbered by the lifetime of a planetary system. Indeed, one can imagine that life in the gas phase might be associated with a galaxy and its energy flux for nearly the age of the universe.
6.3.2
Life in the Solid Phase
Species can diffuse through solids to give chemical reactions.40 Diffusion through the solid phase is slow by terran standards. Nevertheless, given cosmic lengths of time, and the input of energy via cosmic rays or other high-energy particles, a biochemistry able to support Darwinian evolution can certainly be conceived.41 Clathrates and solites, in which different molecules fit into exchangeable cages, might be relevant here.
Allamandola and Hudgins have considered the formation of complex organic species in ice matrices and provided a summary of the photochemical evolution on those ices found in the densest regions of molecular clouds, the regions where stars and planetary systems are formed.42 Ultraviolet photolysis of these ices produces many new compounds, some of which have prebiotic possibilities. These compounds might have played a part in organic chemistry on early Earth.
Given that interstellar ices are the building blocks of comets and comets are thought to be an important source of the species that fell on primitive Earth, the structures of molecules in comets may be related to the origin of life. It is possible that organic materials formed in the solid ice phase of interstellar materials provided raw materials used for life originating solely on Earth. If so, the deep freeze of ice in the Oort cloud would have been an excellent place to store these, especially the unstable ones, awaiting delivery to a planet.
As an alternative, Allamandola and Hudgins considered the possibility that compounds made in ice might have initiated a Darwinian process within the comet. Indeed, a weird life form might reside within solids in the Oort cloud living in deeply frozen water, obtaining energy occasionally from the trail of free radicals left behind by ionizing radiation, and carrying out only a few metabolic transformations per millennium. While such a life form would presumably metabolize slowly, it would not necessarily be constrained by the lifetime of an associated star.
6.4
OPPORTUNITIES FOR RESEARCH
Alternative polar solvents offer potential solutions to paradoxes presented by replicator-first theories of the origin of life. That is particularly true for formamide. Formamide has been shown to be a suitable precursor of
adenine—preferable to hydrogen cyanide—especially in the presence of trace amounts of hydrogen cyanide. Nucleoside phosphates, thermodynamically understandable with respect to hydrolysis in water, can be synthesized in formamide. Furthermore, formamide is a liquid over wide ranges of temperature and pressure; it can form a liquid phase on Mars and can be dehydrated at low pressure, high temperature, or combinations of the two. RNA duplexes need not be unstable in formamide-water mixtures. Small-molecule (metabolism-first) theories of origins avoid such paradoxes but can be expected to be viable with respect to alternative solvents as well as water. That offers an opportunity for a broad research program to explore formamide as a potential prebiotic solvent—a simple task for Earth-based laboratory research.
The discovery of evidence of liquid water-ammonia eutectics on Titan provides a context for the potential for polar fluids outside what is normally regarded as the “habitable zone.” The stay of the Cassini-Huygens mission on the surface of Titan was brief, but this moon of Saturn is the locale that is most likely to support exotic life. The committee believes that it is important to consider whether the planned missions to the solar system should be reordered to permit returning to Titan earlier than now scheduled.
Essentially nothing is known about the solubility of biomolecules in cryogenic solvents. Therefore, research into the possibility of life in cryogenic solvents needs to begin by making fundamental measurements and establishing a database describing the solubility of organic species in such solvents over a range of pressures and temperatures that are relevant to locales in the solar system.
Concepts of life in the gas phase are speculative, but questions related to organic chemistry in ices are experimentally approachable and are components of NASA missions. With sample return from comets imminent, NASA missions have the potential to generate fundamentally new insights into the kinds of organic compounds that might have been present on early Earth. Indeed, the committee concluded that the least expensive mission to the solar system that would have a high potential for fundamentally altering current understanding of how life originated would be the return to Earth of samples of cometary ice. Laboratory studies on Earth can provide sensible simulations of interstellar and solar system ice bodies that would be key to guiding these missions.
6.5
REFERENCES
1 Ward, P.D., and Brownlee, D. 2000. Rare Earth. Why Complex Life Is Uncommon in the Universe. Springer-Verlag, New York.
2 See Kasting, J.F., 2001, Essay review of Peter Ward and Don Brownlee’s Rare Earth: Why Complex Life Is Uncommon in the Universe, Perspect. Biol. Med. 44:117-131.
3 Visser, C.M., and Kellogg, R.M. 1978. Biotin. Its place in evolution. J. Mol. Evol. 11:171-178.
4 Ogren, W.L., and Bowes, G. 1972. Oxygen inhibition and other properties of soybean ribulose 1,5-diphosphate carboxylase. J. Biol. Chem. 247:2171-2176.
5 Brunner, E. 1988. Fluid mixtures at high pressures. 7. Phase separation and critical phenomena in 18 n-alkane ammonia. and 4 n-alkane methanol. mixtures. J. Chem. Thermodyn. 20:1397-1409.
6 Haldane, J.B.S. 1954. The origins of life. New Biology 16:12-27.
7 Olah, G.A., Salem, G., Staral, J.S., and Ho, T.L. 1978. Preparative carbocation chemistry. 13. Preparation of carbocations from hydrocarbons via hydrogen abstraction with nitrosonium hexafluorophosphate and sodium nitrite trifluoromethanesulfonic acid. J. Org. Chem. 43:173-175.
8 Kolodner, M.A., and Steffes, P.G. 1998. The microwave absorption and abundance of sulfuric acid vapor in the Venus atmosphere based on new laboratory measurements. Icarus 132:151-169.
9 Cockell, C.S. 1999. Life on Venus. Planet. Space Sci. 47:1487-1501.
10 Colin, J., and Kasting, J.F. 1992. Venus. A search for clues to early biological possibilities. Pp. 45-65 in Exobiology in the Solar System. NASA-SP-512. NASA, Washington, D.C.
11 Schulze-Makuch, D., and Irwin, L.N. 2004. Life in the Universe: Expectations and Constraints. Springer-Verlag GmbH, Berlin, pp. 128-132.
12 Grinspoon, D.H. 1997. Venus Revealed: A New Look Below the Clouds of Our Mysterious Twin Planet. Perseus Publishing, Cambridge, Mass.
13 Schulze-Makuch, D., and Irwin, L.N. 2002. Reassessing the possibility of life on Venus: Proposal for an astrobiology mission. Astrobiol. 2:197-202.
14 Sagan, C., and Morowitz, H. 1967. Life in the clouds of Venus. Nature 215:1259-1260.
15 Schulze-Makuch, D., Irwin, L.N., and Irwin, T. 2002. Astrobiological relevance and feasibility of a sample collection mission to the atmosphere of Venus. ESA Sp. 518:247-252.
16 Kreuzwieser, J., Schnitzler, J.P., and Steinbrecher, R. 1999. Biosynthesis of organic compounds emitted by plants. Plant Biol. 1:149-159.
17 Schulze-Makuch, D., and Irwin, L.N. 2004. Life in the Universe. Expectations and Constraints. Springer-Verlag GmbH, Berlin.
18 Ricardo, A., Carrigan, M.A., Olcott, A.N., and Benner, S.A. 2004. Borate minerals stabilize ribose. Science 303:196.
19 Schoffstall, A.M. 1976. Prebiotic phosphorylation of nucleosides in formamide. Origins Life Evol. Biosph. 7:399-412.
20 Schoffstall, A.M., Barto, R.J., and Ramo, D.L. 1982. Nucleoside and deoxynucleoside phosphorylation in formamide solutions. Origins Life Evol. Biosph. 12:143-151.
21 Schoffstall, A.M., and Liang, E.M. 1985. Phosphorylation mechanisms in chemical evolution. Origins Life Evol. Biosph. 15:141-150.
22 Sagan, C., Thompson, W.R., and Khare, B.N. 1992. Titan: A laboratory for prebiological organic chemistry. Acc. Chem. Res. 25:286-292.
23 Tawfik, D.S., and Griffiths, A.D. 1998. Man-made cell-like compartments for molecular evolution. Nat. Biotechnol. 16:652-656.
24 West, R.A. 1999. Atmospheres of the giant planets. Encyclopedia of the Solar System. Academic Press, New York.
25 Sagan, C., and Salpeter, E.E. 1976. Particles, environments, and possible ecologies in the Jovian atmosphere. Astrophys. J. 32:737-755.
26 Bains, W. 2004. Many chemistries could be used to build living systems. Astrobiol. 4(2):137-167.
27 Robertson, W.W., and Reynolds, R.E. 1958. Effects of hydrostatic pressure on the intensity of the singlet-triplet transition of 1-chloronaphthalene in ethyl iodide. J. Chem. Phys. 29:138-141.
28 King, A.D., Jr., and Robertson, W.W. 1962. Solubility of naphthalene in compressed gases. J. Chem. Phys. 37:1453-1455.
29 Bains, W. 2004. Many chemistries could be used to build living systems. Astrobiol. 4(2):137-167.
30 Randolph, T.W., Blanch, H.W., Prausnitz, J.M., and Wilke, C.R. 1985. Enzymatic catalysis in a supercritical fluid. Biotechnol. Lett. 7:325-328.
31 Hammond, D.A., Karel, M., Klibanov, A.M., and Krukonis, V. 1985. Enzymatic-reactions in supercritical gases. Appl. Biochem. Biotechnol. 11:393-400.
32 Nakamura, K., Chi, Y.M., Yamada, Y., and Yano, T. 1986. Lipase activity and stability in supercritical carbon-dioxide. Chem. Eng. Commun. 45:207-212.
33 Aaltonen, O., and Rantakyla, M. 1991. Biocatalysis in supercritical CO2.Chem. Tech. 21:240-248.
34 Kamat, S.V., Beckman, E.J., and Russell, A.J. 1995. Enzyme activity in supercritical fluids. Crit. Rev. Biotechnol. 15:41-71.
35 Aaltonen, O. 1999. Enzymatic biocatalysis. Pp. 414-445 in Chemical Synthesis Using Supercritical Fluids (P.G. Jessop and W. Leitner, eds.). Wiley-VCH, New York.
36 Aaltonen, O. 1999. Enzymatic biocatalysis. Pp. 414-445 in Chemical Synthesis Using Supercritical Fluids (P.G. Jessop and W. Leitner, eds.). Wiley-VCH, New York.
37 Rickard, D.T., and Wickman, F.E., eds. 1981. Chemistry and Geochemistry of Solutions at High Temperatures and Pressures: Physics and Chemistry of the Earth, 13/14. Pergamon Press, Oxford.
38 Ikushima, Y., and Arai, M. 1999. Stoichiometric organic reactions. Pp. 259-279 in Chemical Synthesis Using Supercritical Fluids (P.G. Jessop and W. Leitner, eds.). Wiley-VCH, New York.
39 Savage, P.E. 1999. Organic chemical reactions in supercritical water. Chem. Rev. 99:603-621.
40 Huang, B., and Walsh, J.J. 1998. Solid-phase polymerization mechanism of polyethyleneterephthalate affected by gas flow velocity and particle size. Polymer 39:6991-6999.
41 Goldanskii, V.I. 1996. Nontraditional mechanisms of solid-phase astrochemical reactions. Kinet. Catal. 37:608-614.
42 Allamandola, L.J., and Hudgins, D.M. 2003. From interstellar polycyclic aromatic hydrocarbons and ice to astrobiology. Solid State Astrochemistry, NATO Science Series II: Mathematics, Physics, and Chemistry (V. Pirronello and J. Krelowski, eds.). Kluwer, Dordrecht.