2
Environmental Impacts of Genetically Engineered Crops at the Farm Level
The environmental impacts of planting genetically engineered (GE) crops occur within the context of agriculture’s general contribution to environmental change. Agriculture has historically converted biologically diverse natural grasslands, wetlands, and native forests into less diverse agroecosystems to produce food, feed, and fiber. Effects on the environment depend on the intensity of cultivation over time and space; the inputs applied, including water, fertilizer, and pesticides; and the management of inputs, crop residue, and tillage. With 18 percent of the land area in the United States planted to crops and another 26 percent devoted to pastures (FAO, 2008), the huge scale of these impacts becomes obvious. In general, tillage, crop monoculture, fertilizers, and pesticide use often have adverse effects on soil, water, and biodiversity. Agriculture is the leading cause of water-quality impairment in the United States (USDA-ERS, 2006). No-tillage systems, crop rotations, integrated pest management, and other environmentally friendly management practices may ameliorate some of the adverse impacts, but the tradeoff between agricultural production and the environment remains. With agricultural lands approaching 50 percent of U.S. land, developing more ecologically and environmentally sound agricultural management practices for crops, soil, and water is a central challenge for the future (Hanson et al., 2008). Against that backdrop, we evaluate the impact of GE crops on the environmental sustainability of U.S. farms.
This chapter examines the changes in farm practices that have accompanied the adoption of GE crops and the evidence on how such adop-
tion affects the environment. It addresses impacts at the individual farm level and also at the landscape level, given that impacts from individual farms accumulate and affect other farms and their access to communal natural resources in the region. The use of GE crops has altered farmers’ agronomic practices, such as tillage, herbicides, and insecticides; these alterations have implications for environmental sustainability both on and off the farm, which are evaluated to the extent possible at this point in time (Box 2-1). In particular, we examine the effects of the adoption of GE crops on soil quality, biodiversity, and water quality.
ENVIRONMENTAL IMPACTS OF HERBICIDE-RESISTANT CROPS
The adoption of herbicide-resistant (HR) crops has affected the types and number of herbicides and the amount of active ingredient applied to soybean, corn, and cotton. This section first examines the substitution of glyphosate for other herbicides that has taken place and how the use of HR crops has interacted with tillage practices. It then assesses ecological effects of those changes on soil quality, water quality, arthropod biodiversity, and weed communities. Lastly, the implications for weed management in cropping systems with HR crops are considered, especially for systems in which glyphosate-resistant weeds evolve.
Herbicide Substitution
A higher proportion of herbicide-resistant GE soybean has been planted than of any other GE crop in the United States. Adoption has exceeded 90 percent of the acres planted to soybean by U.S. farmers (Figure 2-1). HR cotton acreage reached 71 percent in 2009 (Figure 2-2), while planted HR corn acres were 68 percent that year (Figure 2-3). The HR crops planted thus far have altered the mix of herbicides used in cropping systems and allowed the substitution of glyphosate for other herbicides.1 Figures 2-1 through 2-3 summarize the trends in the use of glyphosate and other herbicides on soybean, cotton, and corn (expressed in pounds of active ingredient per planted acre of these crops) and the adoption of HR soybean, cotton, and corn (Fernandez-Cornejo et al., 2009). It is important to recognize that, depending on the metrics used, the substitution of glyphosate for other herbicides has resulted in the use of fewer alternative herbicides by growers of HR crops. However, glyphosate is often applied in higher doses and with greater frequency than the herbicides it replaced. Thus, the actual amount of active ingredi-
1 |
A list of herbicides for which glyphosate is a common substitute can be found in Appendix A. |
BOX 2-1 Limitations to Evaluating the Magnitude of Environmental Effects Although environmental risk assessment is conducted for all GE varieties before regulatory approval, in some cases the absence of environmental monitoring at the landscape level prevents calculating the magnitude of effects (e.g., water quality) following commercialization. Where monitoring data on agricultural practices are available (e.g., tillage practices, pesticide use), simple correlations of the adoption rates with trends in agricultural practices do not capture the complexity required to quantify the magnitude of any environmental effect. The lack of spatially explicit data linking the use of GE crops with data-monitoring agricultural practices stymies any accurate calculation of the magnitude of environmental effects at national or even regional levels (NRC, 2002). Environmental consequences of agricultural practices can vary greatly at a subregional scale. For example, the adoption of a herbicide-resistant crop may facilitate use of no-till practices, but the environmental effects of no-till practices depend on existing soil texture, structure, and erosion potential for each individual farm. Though models may exist to quantify soil retention given erosion potential, what amount of retention can be attributed to HR crops requires two additional calculations:
Similarly, weed and pest control measures fluctuate from year to year and crop to crop, as have the choices of active ingredients. Determining the extent to which adoption of GE crops replaced specific pesticides over time requires incorporating a suite of factors, such as changes in pest pressure or pest-management strategies (e.g., see footnote on boll weevil eradication program), tillage practices, technology, and public policy (e.g., pesticide regulation, government programs) (Fernandez-Cornejo et al., 2009). Spatial data on the evolution of weed resistance are also lacking, thus preventing any calculation of environmental consequences of the declining effectiveness of glyphosate with glyphosate-resistant crops. |
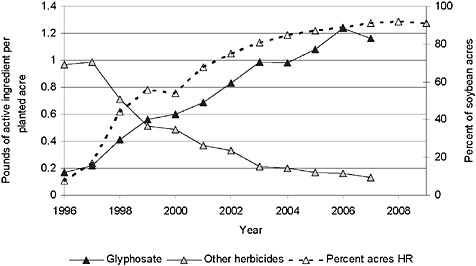
FIGURE 2-1 Application of herbicide to soybean and percentage of acres of herbicide-resistant soybean.
NOTE: The strong correlation between the rising percentage of HR soybean acres planted over time, the increased applications of glyphosate, and the decreased use of other herbicides suggests but does not confirm causation between these variables.
SOURCE: USDA-NASS, 2001, 2003, 2005, 2007, 2009a, 2009b; Fernandez-Cornejo et al., 2009.
ents (glyphosate and other herbicides) applied per acre actually increased from 1996 to 2007 in soybean (Figure 2-1) and cotton (Figure 2-2) but decreased over the same period in corn (Figure 2-3).
Glyphosate is reported to be more environmentally benign than the herbicides that it has replaced (Fernandez-Cornejo and McBride, 2002; Cerdeira and Duke, 2006). It binds to soil rapidly (preventing leaching), it is biodegraded by soil bacteria, and it has a very low toxicity to mammals, birds, and fish (Malik et al., 1989). Glyphosate can be detected in the soil for a relatively short period of time compared to many other herbicides, but is essentially biologically unavailable (Wauchope et al., 1992). Formulations that contain the surfactant polyoxyethylene amine can be toxic to some amphibians at environmentally expected concentrations and may affect aquatic organisms under some environmental conditions (Folmar et al., 1979; Tsui and Chu, 2003; Relyea and Jones, 2009); however, these formulations are labeled for terrestrial uses only with restrictions with respect to waterways. The greater use of postemergence glyphosate applications has been accompanied by modifications of agronomic practices, particularly
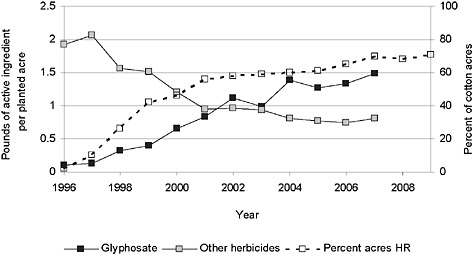
FIGURE 2-2 Application of herbicide to cotton and percentage of acres of herbicide-resistant cotton.
NOTE: The strong correlation between the rising percentage of HR cotton acres planted over time, the increased applications of glyphosate, and the decreased use of other herbicides suggests but does not confirm causation between these variables.
SOURCE: USDA-NASS, 2001, 2003, 2005, 2007, 2009a, 2009b; Fernandez-Cornejo et al., 2009.
with regards to weed management and tillage. The interactions of those practices have implications for environmental sustainability.
Tillage Practices
Tillage is one process used by farmers to prepare the soil before planting. In conventional tillage, all postharvest residue is plowed into the soil to prepare a clean seedbed for planting and to reduce the growth of weeds; in conservation tillage, at least 30 percent of the soil surface is left covered with crop residue after planting. In the 1970s and 1980s, innovations in cultivators and seeders enabled farmers to plant seeds at a reasonable cost with residue remaining on the field. Those developments encouraged the adoption of one form of conservation tillage called no-till, in which the soil and surface residue from the previously harvested crop are left undisturbed as the next crop is seeded directly into the soil without tillage. After soil-conservation policy was incorporated into the Food Security Act of 1985, conservation tillage accelerated in the 1990s. The
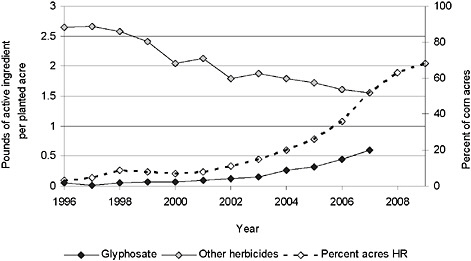
FIGURE 2-3 Application of herbicide to corn and percentage of herbicide-resistant corn.
NOTE: The strong correlation between the rising percentage of HR corn acres planted over time, the increased applications of glyphosate, and the decreased use of other herbicides suggests but does not confirm causation between these variables.
SOURCE: USDA-NASS, 2001, 2003, 2005, 2007, 2009a, 2009b; Fernandez-Cornejo et al., 2009.
introduction of HR soybean and cotton has supported the trend because the use of glyphosate allowed weeds to be controlled after crop emergence without the need for tillage to disrupt weed development before or after planting. Indeed, in the last 10 years, the use of conservation tillage has continued to increase, with the exception that it has remained constant in the case of corn (Figure 2-4).2
The adoption of conservation tillage practices by U.S. soybean growers increased from 51 percent of planted acres in 1996 to 63 percent in 2008, or an addition of 12 million acres. The adoption of no-till practices accounted for most of the increase and was used on 85 percent of these additional 12 million acres. Over the same time period, the acreage planted to soybean increased at most nine million acres. In cotton there was a doubling of the percentage of acres managed using conservation tillage from 1996 to 2008, and no-till is the predominant conservation tillage practice. Cotton acreage declined over the same time period. For
2 |
More information on different types of tillage systems can be found in Appendix B. |
corn between 1996 and 2008, an additional 4.8 million acres of corn were planted. At the same time, the use of conservation tillage practices remained at a fairly constant 40 percent of planted acreage. No-till practices increased by 4 percent over the same time period (4.3 million acres), but this was disproportionate relative to overall increases in conservation tillage practices (1.9 million acres), indicating that farmers converted from other conservation tillage practices to no-till.
According to U.S. Department of Agriculture (USDA) survey data for 1997, a larger share of acreages planted to HR soybean was managed with conservation tillage than was planted to conventional soy-bean (Fernandez-Cornejo and McBride, 2002)—about 60 percent versus about 40 percent (Figure 2-5). The difference in the use of no-till between adopters and nonadopters of HR soybean was even more pronounced: 40 percent of acres planted with HR soybean were under no-till, double the corresponding share of acres of non-GE soybean under no-till management practices (Fernandez-Cornejo and McBride, 2002).
From the perspective of farmer decision making, the availability of herbicide-resistance technology may affect the adoption of conservation tillage, and the use of conservation tillage may affect the decision to adopt HR crops. Several economists have tried to understand how closely the two decisions are linked. An econometric model developed to address the simultaneous nature of the decisions was used to determine the nature of the relationship between the adoption of GE crops with HR traits and no-till practices on the basis of 1997 national survey data on soybean farmers (Fernandez-Cornejo et al., 2003). Farmers using no-till were found to have a higher probability of adopting HR cultivars than farmers using conventional tillage, but using HR cultivars did not significantly affect no-till adoption. That result suggested that farmers already using no-till incorporated HR cultivars seamlessly into their weed-management program; but the commercialization of HR soybean did not seem to encourage the adoption of no-till, at least at the time of the survey.
More recently, however, Mensah (2007) found a two-way causal relationship on the basis of more recent data. Using a simultaneous-adoption model and 2002 survey data on soybean farmers, Mensah found that farmers who adopted no-till were more likely to adopt HR soybeans and that farmers who adopted herbicide-resistance technology were more likely to adopt no-till practices.
In the case of cotton, the evidence also points toward a two-way causal relationship. Roberts et al. (2006) evaluated the relationship between adoption of HR cotton and conservation tillage practices in Tennessee from 1992 to 2004. Using two methods,3 they found that the adoption of
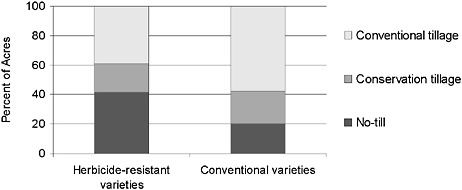
FIGURE 2-5 Soybean acreage under conventional tillage, conservation tillage, and no-till, 1997.
SOURCE: Adapted from Fernandez-Cornejo and McBride, 2002.
HR cotton increased the probability that farmers would adopt conservation tillage and conversely that farmers that had previously adopted conservation tillage practices were more likely to adopt HR cotton. Thus, the adoption of no-till and the adoption of HR cotton are complementary practices.
Kalaitzandonakes and Suntornpithug (2003) also studied the simultaneous adoption of HR and stacked cotton varieties and conservation tillage practices on the basis of farm-level data. They concluded that conservation tillage practices both encouraged the adoption of HR and stacked cotton varieties and were encouraged by their adoption. Using state-level data for 1997–2002 and using a simultaneous-equation econometric model, Frisvold et al. (2007) studied the diffusion of HR cotton and conservation tillage. They found strong complementarity between the two practices and rejected the null hypothesis that the diffusion of one is independent of the diffusion of the other. They also observed that an increase in the probability of adoption of HR cotton increased the probability of adoption of conservation tillage and vice versa.
Thus, most empirical evidence points to a two-way causal relationship between the adoption of HR crops and conservation tillage.4 Farmers using conservation tillage practices are more likely to adopt HR crop varieties than those using conventional tillage, and those adopting HR crop varieties are more likely to change to conservation tillage practices than those who use non-HR cultivars. The analytical techniques used do
not reveal the relative strength of each causal linkage, so it is not clear which factor (adoption of HR varieties or use of conservation tillage) has a greater influence on the other.
Soil Quality
The relationship between the adoption of conservation tillage practices and the adoption of HR crops is relevant to farm sustainability because conservation tillage has fewer adverse environmental impacts than conventional tillage (reviewed by Uri et al., 1999). On the farm, conservation tillage reduces soil loss from erosion, increases water infiltration, and can improve soil quality and moisture retention (reviewed by Uri et al., 1999; Holland, 2004). Corn and soybean are grown in regions where highly erodible land is common, and conversion to conservation tillage for these crops results in substantial reduction in soil loss and wind erosion even on non-highly erodible land (Uri et al., 1999). Leaving more crop residue on fields strengthens nutrient cycling and increases soil organic matter, a key component of soil quality (reviewed by Blanco-Canqui and Lal, 2009). Soil organisms decompose plant residue, and this, in turn, cycles nutrients and improves soil structure. In general, soil organisms have greater abundance or biomass in no-till systems than in conventional tillage systems because soil is disturbed less (reviewed by Wardle, 1995; Kladivko, 2001; Liebig et al., 2004).
In addition to tillage, the use of herbicides can affect soil quality through their impact on soil organisms, so interpreting the effects of HR crops on soil quality requires an understanding of how tillage practices interact with herbicide use to influence the soil microorganism community. In laboratory studies, glyphosate can inhibit or stimulate microbial activity, depending on soil type and glyphosate formulation (Carlisle and Trevors, 1986, and references therein). Some microorganisms can use glyphosate as a substrate for metabolism (increased activity), whereas others are susceptible to the herbicide because they have an enzyme 5-enolpyruvyl-shikimate-3-phosphate synthase pathway that glyphosate inhibits. When species-level responses were measured, roots of glyphosate-resistant soybean and corn treated with glyphosate had significantly more colonies of the fungus Fusarium than did non-HR cultivars or HR cultivars not treated with glyphosate (Kremer and Means, 2009). In contrast, fluorescent Pseudomonas populations, an antagonist of fungal pathogens like Fusarium, were significantly lower in soybean that were both glyphosate resistant and treated with glyphosate compared to untreated HR cultivars or a non-HR cultivar treated with other herbicides (Kremer and Means, 2009). Those results indicate a change in the antagonistic relationship between Fusarium and Pseudomonas attributable
to the formulation of glyphosate used. Whether magnitude of change in this antagonistic relationship would have consequences on soil quality of disease control was not a part of the study.
With respect to general microbial activity, three studies in the United States have detected no uniform changes in soil organism profiles in association with tillage or with the use of glyphosate on glyphosate-resistant cropping systems (Liphadzi et al., 2005; Weaver et al., 2007; Locke et al., 2008). Soil microorganisms in fields planted with glyphosate-resistant corn and soybean varieties were similar with and without tillage (Liphadzi et al., 2005). HR fields treated with glyphosate and non-GE fields treated with other herbicides were also similar in soil microbe activity (Liphadzi et al., 2005). On tilled, experimental plots of glyphosate-resistant soybean, transient changes in the soil microbial community were detected in the first few days after application of glyphosate compared to no application (Weaver et al., 2007), but the differences disappeared after 7 days. When there was continuous cotton cropping, soil quality did not differ between HR and non-HR systems. In contrast, soil under continuous HR-corn cropping contained more carbon and nitrogen than soil with non-HR corn (Locke et al., 2008), which would be considered a benign change. Differences in carbon and nitrogen contents could have been due to glyphosate use, but they were also probably influenced by changes in the detrital food web associated with the higher biomass of winter weeds in the HR-corn cropping system (Locke et al., 2008). Subtle differences in the structure of the soil microbial community were also detectable in those same experiments; the significance of the differences for soil quality were not discussed. Thus, species-level studies suggest that glyphosate can alter the microbial composition in the rhizosphere. General studies of the interaction of tillage and glyphosate use in HR crops have indicated transient benign effects of glyphosate and neutral, or in one case favorable, effects of conservation tillage on the soil communities in HR crops.
Water Quality
Conservation tillage practices can have off-farm benefits for water quality that are potentially more important than onsite productivity effects (Foster and Dabney, 1995). Because conservation tillage practices improve soil-water infiltration, the volume of runoff is less than when conventional tillage is used. Reduced tillage and no-till practices can improve water quality by reducing the amounts of sediments and sediment-associated chemicals in runoff from farm fields into surface water. Similarly, lower volumes of runoff can decrease the transport of soil nutrients and agricultural inputs, such as fertilizers and pesticides, although the decrease will vary with soil type, tillage practice, and nutrient or pesticide input.
For example, although the concentration of herbicide in runoff from no-till fields can be higher than when other conservation tillage practices are used, the total amount of herbicide in runoff may be similar because runoff volume is reduced (Fawcett et al., 1994; Locke and Bryson, 1997; Mickelson et al., 2001; Shipitalo and Owens, 2006; Zeimen et al., 2006). That phenomenon has been observed with the use of glyphosate in no-till fields (Shipitalo et al., 2008).
Studies have suggested that the use of glyphosate poses less risk to water quality than the use of other herbicides; this is attributable in part to the production systems typically used in GE crops and to the physical chemistry and relatively low toxicity of glyphosate (Estes et al., 2001; Wauchope et al., 2002; Peterson and Hulting, 2004). However, there are no regional-scale analyses of the effects of HR-crop adoption on water quality. One study conducted in a small Ohio watershed that compared herbicide runoff in HR and non-HR soybean fields found that the amount of glyphosate in the runoff was nearly one-seventh that of the herbicide metribuzin and about half that of alachlor, even though glyphosate was applied to soybean twice and alachlor and metribuzin once to soy-bean (Shipitalo et al., 2008). Those results are consistent with known characteristics of glyphosate, which strongly absorbs to soil and has a half-life in soil of 6–60 days, depending on soil characteristics. Microbial processes degrade glyphosate into two metabolites: sarcosine and aminomethylphosphonic acid (AMPA). Sarcosine degrades quickly to carbon dioxide and ammonia. AMPA is more persistent than glyphosate in the soil environment but is considered equally or less toxic (reviewed by Giesy et al., 2000). Numerous studies have documented the occurrence of glyphosate and AMPA in surface waters (Kolpin et al., 2006), but they have rarely been found in groundwater (Borggaard and Gimsing, 2008). Concentrations of glyphosate reported in surface water have not exceeded the maximum contaminant level (MCL) for drinking water set by the U.S. Environmental Protection Agency (EPA); in accordance with World Health Organization recommendations, MCLs have not been set for AMPA (WHO, 2005).
Shifts to conservation tillage attributable to the availability of HR crops have contributed to reductions in soil loss and probably in herbicide runoff. The magnitude and spatial distribution of the benefits are not precisely known, but the implications are that those are important environmental benefits of these cropping systems. However, as discussed later in this chapter (see “Other Shifts in Weed Communities”), some of the environmental benefits may be threatened in the future.
Arthropod Biodiversity
Changes in herbicide use and tillage practices like those accompanying the adoption of HR crops can affect such organisms as natural enemies of pests or pollinators, which provide ecological services to agriculture. Weeds provide an ecological disservice to farms by competing with crop plants for nutrients and light even at low population densities, but weeds can also support a broad array of nonpest species. Pollinators feed on nectar or use some weeds as hosts for their larval stage; weed species can be food for herbivores that in turn are preyed on by predators that also control pests of crops. In particular, more effective weed management could decrease the abundance of beneficial organisms, depending on the mobility of a species and how closely its resource base is associated with weed abundance. In contrast, the increase in no-till practices that leave more plant material undisturbed in fields may increase the resource base for beneficial insects.
Evidence indicates that the planting of HR cultivars does not consistently affect the weed diversity and abundance that support beneficial species. Whether a farmer used a GE crop or a conventional crop, better weed control has generally reduced the numbers of arthropods and other organisms in corn, sugar beet, and rapeseed fields (Hawes et al., 2003) and decreased the abundance of the predatory big-eyed bug (Geocoris punctipes) in soybean fields (Jackson et al., 2003; Jackson and Pitre, 2004). When HR crops improved weed management (decreased weeds), populations of natural enemies and pollinators decreased (Hawes et al., 2003). When conventional weed-management tactics (such as the use of the herbicide atrazine) were more effective at weed control on non-HR corn relative to HR corn, beneficial insect abundance was greater within the HR side of the field where more weeds occurred (Hawes et al., 2003). Subsequent analyses of these same data in more depth have revealed detailed associations between properties of the weed community and the accompanying arthropod food web (Hawes et al., 2009) and strengthened the conclusion that weed management accounts for the relationships observed. However, weed management was not the largest influence on the abundance of beneficial organisms. Rather, there were differences of a factor of 3–10 in abundance among different crops and between early and late in the growing season, compared with differences of a factor of 2 associated with weed management (Hawes et al., 2003).
Weed Biodiversity and Weed Shifts
Crop-production practices inevitably influence the composition of the weed community. Typically, only a few weed species are economically important in a particular crop-production system (Owen, 2001; Tuesca
et al., 2001). When a production practice changes, for example, a change in herbicide, it may ultimately select for weed biotypes that are resistant to that herbicide (Baker, 1991). Other elements of production practices that have selective effects on the weed community include harvesting techniques, irrigation, fertilization, planting dates, soil amendments, and tillage (Hilgenfeld et al., 2004; Murphy and Lemerle, 2006; Owen, 2008).
The stronger the selective force of those practices (e.g., the level of disturbance caused by tillage), the more consistent the selective force (e.g., continuous planting of the same crop as opposed to annual crop rotations), and the simpler the selective force (e.g., the recurrent use of one herbicide), the greater the effect on the composition of the weed community (Owen, 2001). Changes in the kinds of weeds that are important locally are termed weed shifts (which implies changes in weed species composition) (Givnish, 2001); in the following discussion, weed shifts are the ecological process by which an initial weed community is replaced by a new community, including better-adapted species, in response to changes in agricultural practices. Weed shifts are generally followed by a period of stability, given the longevity of weed seeds in the soil, as long as the agricultural systems that resulted in the shift remain constant (Buhler, 1992; Buhler et al., 1997). They are a common and inevitable result of agriculture and are not unique to the adoption of HR crops, but it is essential to understand and manage them well if agriculture is to be productive and sustainable. Such shifts are particularly relevant for managing weeds. in HR-crop systems, in which tillage practices and herbicide use both play major roles in shaping the weed community.
Herbicide Resistance in Weeds
The International Survey of Herbicide Resistant Weeds (ISHRW) provides a historical account and extensive list of weeds that have evolved resistance to herbicides (Heap, 2010). Although the ISHRW reflects the efforts of many weed scientists in reporting weed populations that have herbicide resistance, the voluntary basis of the contributions likely results in underestimation of the extent of resistance to herbicides, including glyphosate. The evolution of herbicide-resistant weeds is not unique to the herbicides for which HR traits exist. Currently, 195 species (115 dicots and 80 monocots) have evolved resistance to at least one of 19 herbicide mechanisms of action in at least 347 herbicide-resistant weed biotypes distributed over 340,000 fields (Heap, 2010).
Glyphosate, first commercialized in 1974, has been extensively used for weed control in perennial crops (fruits, trees, nuts, and vines), along roadsides and irrigation canal banks, and in urban areas and national parks (Powles, 2008). The first case of evolved resistance to glyphosate was reported in 1996 in rigid ryegrass (Lolium rigidum) (Powles et al.,
1998). The glyphosate-resistant population originated in an orchard in a large winter cropping region of southern Australia, where glyphosate had been used intensively for the control of rigid ryegrass for more than 15 years. Since the initial report, at least six other weed species have been reported as resistant to glyphosate in environments where glyphosate-resistant crops were not planted (Powles, 2008; Heap, 2010).
Emergence of Glyphosate-Resistant Weeds in Herbicide-Resistant Crop Fields
Ten species have evolved resistance to glyphosate independently in glyphosate-resistant crops over 14 years in the United States (from 1996 to 2010) (Heap, 2010). Gene flow between HR crops and closely related weed species does not explain the evolution of glyphosate resistance in U.S. fields because sexually compatible weeds are absent where corn, cotton, and soy-bean are grown in the United States. However, the nearly exclusive reliance on glyphosate for weed control, a practice accelerated by the widespread introduction of glyphosate-resistant crop varieties, has caused substantial changes in weed communities. The first report of glyphosate resistance associated with a GE glyphosate-resistant crop involved horseweed (Conyza canadensis) in Delaware (VanGessel, 2001); once resistance evolved, growers found it difficult to control this weed in no-till glyphosate-resistant soy-bean (VanGessel, 2001). Since the initial report in 2000, glyphosate-resistant populations of horseweed have been documented throughout the Mid-Atlantic, Mid-South, Mississippi Delta, and Midwest states (Heap, 2010). The weed grows particularly well in no-till production systems, producing a large number of wind-carried seeds that are dispersed over long distances (Buhler and Owen, 1997; Ozinga et al., 2004).
Subsequent to that discovery in 2000, other weed species have evolved resistance to glyphosate in glyphosate-resistant crops in the United States (Table 2-1). They include two species of pigweed, Palmer amaranth (Amaranthus palmeri) and waterhemp (Amaranthus tuberculatus),5 which have become economically important in glyphosate-resistant cotton and soybean production (Zelaya and Owen, 2000, 2002; Culpepper, 2006; Culpepper and York, 2007; Legleiter and Bradley, 2008). Infested areas are increasing rapidly in the Southeast, the Mississippi Delta (Palmer amaranth as well as Johnsongrass, Sorghum halepense), and the Midwest (waterhemp) (Culpepper and York, 2007; Legleiter and Bradley, 2008). Glyphosate-resistant populations of giant ragweed (Ambrosia trifida) have been reported in several states (Leer, 2006), primarily in or adjacent to glyphosate-resistant soybean. Kochia (Kochia scoparia) with evolved resistance to glyphosate has recently been identified in Kansas (Heap, 2010).
TABLE 2-1 Weeds That Evolved Resistance to Glyphosate in Glyphosate-Resistant Crops in the United States
Species |
Crop |
Location |
Acreagea |
Amaranthus palmeri (Palmer amaranth) |
Corn, cotton, soybean |
Georgia, North Carolina, Arkansas, Tennessee, Mississippi |
200,000–2,000,000 |
Amaranthus tuber culatus (waterhemp) |
Corn, soybean |
Missouri, Illinois, Kansas, Minnesota |
1,200–11,000 |
Ambrosia artemisiifolia (common ragweed) |
Soybean |
Arkansas, Missouri, Kansas |
<150 |
Ambrosia trifida (giant ragweed) |
Cotton, soybean |
Ohio, Arkansas, Indiana, Kansas, Minnesota, Tennessee |
2,000–12,000 |
Conyza canadensis (horseweed) |
Corn, cotton, soybean |
14 states |
> 2,000,000 |
Kochia scoparia (kochia) |
Corn, soybean |
Kansas |
51–100 |
Lolium multiflorum (Italian ryegrass) |
Cotton, soybean |
Mississippi |
1000–10,000 |
Sorghum halepense (Johnsongrass) |
Soybean |
Arkansas |
Unknown |
aMinimum and maximum acreages are based on expert judgments provided for each state. The estimates were summed and rounded to provide an assessment of the minimum and maximum acreages in the United States. These values indicate orders of magnitudes but do not provide precise information on abundance of resistant weeds. SOURCE: Data from Heap, 2010. |
Another weed, common lambsquarters (Chenopodium album) (Kniss et al., 2004, 2005; Schuster et al., 2007; Scursoni et al., 2007) may have also evolved glyphosate-resistant biotypes (Boerboom, 2005), but it has not yet appeared on the ISHRW list.
Other Shifts in Weed Communities
Factors other than the evolution of glyphosate resistance affect the composition of weed species in the field. Changes in the tillage system used in growing HR crops are probably the most important factor in promoting weed shifts because disturbance is a primary selective force (Buhler, 1992). In addition, weeds that escape glyphosate applications by germinating after the last application can have an advantage in glyphosate-resistant crops (Hilgenfeld et al., 2004; Owen and Zelaya, 2005; Puricelli and Tuesca, 2005; Scursoni et al., 2007; Wilson et al., 2007; Owen, 2008). Table 2-2 lists weed species that have been found to be naturally tolerant to the conditions prevalent in the fields where glyphosate-resistant crops are grown and have become more abundant after the widespread adoption of these crops. Shifts in local weed communities have been observed more frequently in glyphosate-resistant cotton and soybean than in glyphosate-resistant corn, probably because glyphosate-resistant cotton and soybean are more widely cultivated than glyphosate-resistant corn (Culpepper, 2006). However, where glyphosate-resistant corn and glyphosate-resistant soybean are commonly rotated (e.g., in the Midwest), strong selection pressure exists for the evolution of glyphosate-resistant weeds because the management tactics vary so little between the two crops.
Farmers’ Response to Glyphosate Resistance in Weeds
The evolution of glyphosate resistance in some kinds of weeds and other weed shifts can diminish the technical and economic efficiency of weed control. However, because glyphosate allows producers to control a wide array of weeds conveniently and economically, they have been reluctant to stop using glyphosate-resistant crops and glyphosate when facing control problems arising from a few glyphosate-resistant or naturally glyphosate-tolerant weed species. For controlling problematic weeds, they prefer increasing the magnitude and frequency of glyphosate applications, using other herbicides in addition to glyphosate, or increasing their use of tillage.
For example, soybean growers in Delaware continued planting glyphosate-resistant soybean even in the presence of widespread glyphosate resistance in horseweed (Scott and VanGessel, 2007). Most producers addressed the problem by applying an herbicide with a different mode of
TABLE 2-2 Weeds Reported to Have Increased in Abundance in Glyphosate-Resistant Crops
Species |
Crop |
Location |
Reference |
Acalypha spp. (copperleaf) |
Soybean |
— |
Owen and Zelaya, 2005; Culpepper, 2006 |
Amaranthus tuberculatus (waterhemp) |
Soybean |
— |
Owen and Zelaya, 2005 |
Amaranthus palmeri (Palmer amaranth) |
Cotton |
— |
Culpepper, 2006 |
Annual grasses |
Cotton |
— |
Culpepper, 2006 |
Chenopodium album (common lambsquarters) |
Soybean |
Iowa, Minnesota |
Owen, 2008 |
Commelina communis (Asiatic dayflower) |
Cotton, soybean |
Midwest, Midsouth, Southeast |
Owen and Zelaya, 2005; Culpepper, 2006; Owen, 2008 |
Commelina benghalensis (tropical spiderwort) |
Cotton |
Southeast, Georgia |
Owen, 2008; Mueller et al., 2005 |
Cyperus spp. (nutsedge) |
Cotton |
— |
Culpepper, 2006 |
Equisetum arvense (field horsetail) |
Herbicide-resistant crops |
— |
Owen, 2008 |
Oenothera biennis (evening primrose) |
Herbicide-resistant crops |
Iowa |
Owen, 2008 |
Oenothera laciniata (cutleaf evening primrose) |
Soybean |
— |
Culpepper, 2006 |
Pastinaca sativa (wild parsnip) |
Herbicide-resistant crops |
Iowa |
Owen, 2008 |
Phytolacca americana (pokeweed) |
Herbicide-resistant crops |
— |
Owen, 2008 |
Ipomoea spp. (annual morning glory) |
Cotton |
— |
Culpepper, 2006 |
action, increasing the frequency of glyphosate applications, or using tillage before planting. Some 76 percent of growers estimated that resistance in horseweed increased their management costs by more than $2.02/acre, and 28 percent reported cost increases of over $8.09/acre (Scott and VanGessel, 2007). Similarly, a survey of 400 corn, soybean, and cotton producers in 17 states found that most would not limit the use of glyphosate-resistant crops when facing problematic glyphosate-resistant weeds (Foresman and Glasgow, 2008). Instead, producers planned to increase the rotation of herbicides, the use of tank-mixes, or the amount of tillage. They expected that additional measures for the control of glyphosate-resistant weeds would cost $13.90–16.30/acre (Foresman and Glasgow, 2008).
In an economic analysis of weed-management costs with a hypothetical reduction of control with glyphosate in three regions of the United States, the projected cost of new resistance-management practices for horseweed was $12.33/acre in a cotton–soybean–corn rotation in western Tennessee (Mueller et al., 2005). Additional costs were due to a shift from no-till to conventional tillage for cotton and the need for new preplant herbicides for soybean. The projected cost of new herbicide resistance-management practices for waterhemp was $17.91/acre in a corn–soybean rotation in southern Illinois; this cost resulted from use of different preemergence and postemergence herbicides for soybean (Mueller et al., 2005). For cotton grown in Georgia, the extra cost of controlling shifts in tropical spiderwort (Commelina benghalensis), a weed that is naturally tolerant to glyphosate, was predicted to be $14.91/acre; an additional herbicide application after cotton emergence explained this cost (Mueller et al., 2005).
Those studies indicate that the evolution of glyphosate resistance and weed shifts could lead to two important changes in practices: increased use of herbicides generally and reductions in conservation tillage (Mueller et al., 2005). Such changes would also increase weed-management costs and reduce producers’ profits, and the environmental consequences of those practices, if they were widely adopted by producers of HR crops, would negate the environmental benefits previously achieved.
In summary, most glyphosate-resistant weeds in HR crops are of economic importance in row crops grown in the Southeast and Midwest. The number of weed species evolving resistance to glyphosate is growing (Figure 2-6), and the number of locations with glyphosate-resistant weeds is increasing at a greater rate, as more and more acreage is sprayed with glyphosate. Though the number of weeds with resistance to glyphosate is still small compared to other common herbicides,6 the shift toward
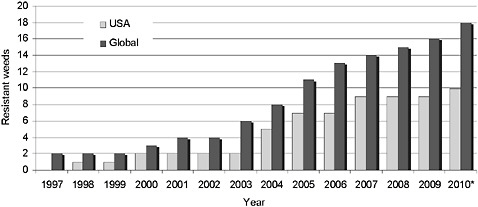
FIGURE 2-6 Number of weeds with evolved glyphosate resistance.
*Weed numbers are updated through March 2010.
SOURCE: Adapted from Heap, 2010.
glyphosate-resistant weed biotypes will probably become an even more important component of row-crop agriculture unless production practices (such as recurrent use of glyphosate) change dramatically (Gressel, 1996; Owen and Zelaya, 2005; Johnson et al., 2009).
Implications of Weed Shifts in Herbicide-Resistant Cropping Systems
As noted above, because the adoption of HR crops has facilitated an increase in conservation tillage and reduced the number of herbicides that growers use to control weeds, the selection pressures affecting weed communities has changed. Unsurprisingly, managing weeds through glyphosate applications to HR crops favors the evolution of glyphosate resistance in weeds occurring in these crop fields (Shaner, 2000; Mueller et al., 2005; Foresman and Glasgow, 2008; Powles, 2008). Addressing the problem of resistance—a problem not unique to HR crops—requires careful thought about management practices and other potential solutions based on a clear understanding of how genes that code for resistance are distributed throughout a population of a weed species.
Principles of Population Genetics Underlying Resistance in Weeds
Similar concepts have been used to understand the evolution of resistance to glyphosate in weeds and to the Bt toxin in insects. Population-genetic models and empirical data on factors that affect how resistance evolves have been applied to the management of both herbicide-resistant
weeds and Bt-resistant insects (Jasieniuk et al., 1996; Werth et al., 2008). However, strategies for delaying the evolution and spread of resistance are not the same because there are important underlying differences in the population genetics of herbicide resistance and insect resistance.
Resistance to herbicides, and in particular to glyphosate, is often conferred by a single nuclear gene (Jasieniuk et al., 1996; Powles and Preston, 2006). Herbicide resistance in weeds is rarely recessive;7 in all cases studied, resistance to glyphosate was additive to dominant, that is, individuals with a single resistance allele can survive applications of glyphosate (Jasieniuk et al., 1996; Zelaya et al., 2004; Powles and Preston, 2006; Zelaya et al., 2007; Neve, 2008). Furthermore, even if resistance is recessive in some weeds, many weeds are self-pollinating, so a recessive gene for resistance could become homozygous in only a few generations and thus confer resistance to all offspring (Gould, 1995; Jasieniuk et al., 1996). Some agronomically important weeds (such as pigweed) are dioecious (having separate male and female plants) and thus are cross-pollinated. They have demonstrated the ability to evolve resistance to glyphosate although the genetics of the process have not been described.
Finally, even though the seeds of some weed species can disperse over long distances (Shields et al., 2006), dispersal of viable pollen generally occurs over short distances (Jasieniuk et al., 1996; Roux et al., 2008). Therein lies an important difference between weeds and insects and hence the availability of strategies, such as refuges, to control weed resistance. For all the reasons described above, maintaining a refuge—an area where susceptible weeds are not exposed to glyphosate and would persist to interbreed with resistant biotypes—cannot be expected to lower the heritability of herbicide resistance in weeds as it lowers the heritability of Bt resistance in insects targeted by Bt crops (Jasieniuk et al., 1996). The refuge strategy for Bt-resistant insects is discussed later in this chapter (see “Evolution and Management of Insect Resistance”).
Although the use of refuges cannot be expected to delay the evolution of glyphosate resistance in weeds, the spread of herbicide resistance can be delayed by reducing the selective differential (the difference in survival and other fitness traits) between individuals with and without resistance alleles (Gressel and Segel, 1990; Jasieniuk et al., 1996; Werth et al., 2008). That can be accomplished by using control practices that kill weeds that
have the resistance alleles. For example, the use of tank-mixes that contain two or more herbicides with different modes of action may be effective if the herbicides have high efficacy in controlling the target weeds. Similarly, herbicides with different modes of action or methods that combine herbicides and mechanical weed control (tillage) may be used sequentially to control the same generation (i.e., emergence cohort) of weeds.
The selective differential between individuals with and without resistance alleles can also be reduced by rotating the types of herbicides used to control the target weeds so that selection for resistance to a specific herbicide occurs only in alternate growing seasons (Jasieniuk et al., 1996; Roux et al., 2008). When no fitness costs8 are associated with resistance, the rotation of herbicides contributes to equalizing the fitness of individuals that are resistant to and susceptible to a herbicide during seasons when the herbicide is not used. Models suggest that the evolution of resistance to the rotated herbicides will be delayed by 1 year for each year that the rotation tactic is used (Maxwell and Jasieniuk, 2000). When fitness costs are associated with resistance to a herbicide (Gressel and Segel, 1990; Jasieniuk et al., 1996; Baucom and Mauricio, 2004), the fitness of individuals that have resistance alleles is lower than the fitness of individuals that do not during seasons when the herbicide is not used. Therefore, herbicide rotation contributes to reducing the selective differential between individuals with and without resistance alleles over time, which may delay the evolution of resistance (Jasieniuk et al., 1996; Roux et al., 2008).
Reduction in the selective differential can be accomplished by rotating the type of crops grown in a field between growing seasons; this may result in drastic changes in the types of herbicides used. Changes in ecological conditions associated with cultivation of different crops could favor declines in particular weed species (which could be resistant or tolerant to glyphosate) or induce competitive disadvantages in herbicide-resistant weeds through negative cross-resistance, in which resistance to one chemical confers hypersensitivity to another chemical (Gressel and Segel, 1990; Boerboom, 1999; Owen and Zelaya, 2005; Beckie et al., 2006; Murphy and Lemerle, 2006).
Developing Weed-Management Strategies for Herbicide-Resistant Crops
How might the various strategies be used in the context of HR cropping systems? Tank-mixes and sequences of herbicides to extend the useful life of herbicides could be employed if crop cultivars that are resistant to two or more herbicides are developed; this strategy is currently favored by biotechnology companies (Duke, 2005; Behrens et al., 2007; Green et al., 2008; Green, 2009). As for using crop rotations, the increasingly common practice of farmers throughout the United States of using glyphosate as the primary or only weed-management tactic in rotations of different glyphosate-resistant crops limits the application of the rotation strategy, even if the change in crop-induced ecological changes might improve weed management. A possible solution could be to combine the rotation of two or more HR cultivars that each can tolerate only one herbicide with the use of a different herbicide at each rotation. For example, different varieties of GE canola (Brassica napus L.) grown in the prairie provinces of Canada were engineered for resistance to glufosinate or glyphosate. That allowed producers to include two types of HR canola into a canola–wheat–barley rotation so that canola resistant to glufosinate or glyphosate would be grown only once every 4 years in a particular field (Powles, 2008). In contrast with corn, soybean, and most cotton production, growing crop species like canola, in which hybridization between the crop and weedy relatives is possible, poses a risk of gene flow between the HR crop and the weedy relatives (Beckie et al., 2003; Légère, 2005; see also “Gene Flow Between Genetically Engineered Crops and Related Weed Species”).
The same rotation strategy could be used with HR crops that are resistant to two (or more) herbicides; the same crop would be grown twice during the rotation cycle, but each of the two herbicides that it can resist would be applied only every other year. Genes that confer resistance to some acetyl-CoA carboxylase (ACCase) inhibitors, synthetic auxins (e.g., 2, 4-D), acetolactate synthase (ALS) inhibitors, dicamba, glufosinate, glyphosate, and hydroxyphenylpyruvate dioxygenase (HPPD) inhibitors are the most likely candidates for production of the next generation of HR varieties that are resistant to multiple herbicides (Duke, 2005; Behrens et al., 2007; Green, 2009). So far, weed resistance to glufosinate and HPPD inhibitors has not been reported. Weed resistance to dicamba has not been reported in corn, cotton, or soybean but has appeared in other crops in the United States (Heap, 2010). However, weed resistance to some ACCase inhibitors, synthetic auxins, and ALS inhibitors has been reported in corn, cotton, and soybean (Heap, 2010). Moreover, most weed species that have evolved resistance to glyphosate in fields of HR crops (Table 2-1) also have evolved resistance to ALS inhibitors (Heap, 2010).
From the point of view of herbicide-resistance management and the long-term efficacy of an HR crop, it may be better to engineer a crop for
resistance to herbicides that can efficiently control most weeds associated with the crop. For example, genes that confer resistance to ALS inhibitors, to which many weed species are already resistant, could be inferior to genes that confer resistance to dicamba, glufosinate, and HPPD inhibitors to produce durable HR corn, cotton, and soybean resistant to two or more herbicides. Similarly, care should be taken to engineer crops for resistance to specific ACCase inhibitors and synthetic auxins that will still be effective in controlling weeds associated with future HR crops.
If crops that are resistant to multiple herbicides—including ALS inhibitors, ACCase inhibitors, synthetic auxins, and glyphosate—are widely planted, continued use of the herbicides in fields that contain weeds already resistant to some of them could involve a risk of selecting for high levels of multiple herbicide resistance. The ability of weeds to evolve biotypes that have multiple herbicide resistance has already been demonstrated in waterhemp populations in Illinois and Missouri that are resistant to three herbicide mechanisms of action (Patzoldt et al., 2005; Legleiter and Bradley, 2008). Evolved multiple resistance will exacerbate problems of controlling some key herbicide-resistant weeds, and local and regional spatially explicit information on the distribution of weeds that are resistant to glyphosate and other herbicides could be useful in helping to manage such a situation (Werth et al., 2008). Tank-mixes and sequencing herbicides rely on redundancy to be effective. Models assessing sequential use of herbicides only, or of herbicides and mechanical weed control, indicate that a low frequency of alleles conferring resistance to herbicides and high weed mortality are critical factors for these strategies to substantially delay the evolution of weed resistant to glyphosate in HR crops (Neve et al., 2003; Neve, 2008; Werth et al., 2008).
In conclusion, regardless of the specific herbicide for which HR crops are genetically engineered, only appropriate stewardship by the grower will delay the evolution of resistance to the herbicide. Resistance management is voluntary in the United States for all pesticides except Bt produced by Bt crops (Berwald et al., 2006; Thompson et al., 2008). Given the rapid increase in and expansion of weeds that are resistant to glyphosate in HR crops, herbicide-resistance management needs national attention. As discussed previously, the rapid evolution of weed resistance to glyphosate has probably been a consequence of growers’ management decisions that favored the use of glyphosate as the primary, if not sole, tactic to control weeds despite efforts in the private and public sectors to strongly recommend alternative strategies (Johnson et al., 2009). Without changes in production practices, the increase in weeds resistant to glyphosate will likely increase weed-management expenses for farmers. The evolution of herbicide resistance and other weed shifts associated with the adoption of GE crops requires the development and use of more
effective weed-management strategies and tactics (Beckie, 2006; Murphy and Lemerle, 2006; Green et al., 2008; Gustafson, 2008; Powles, 2008; Werth et al., 2008).
Diversification of weed-management strategies can be accomplished by integrating several weed-control tactics: herbicide rotation, herbicide application sequences, and the use of tank-mixes of more than one herbicide; the use of herbicides that have different modes of action, methods of application, and persistence; cultural and mechanical control practices; and equipment-cleaning and harvesting practices that minimize the dispersal of herbicide-resistant weeds. Although the strategies to mitigate weed shifts are readily identified, they have largely been ignored because of the scale of commercial agriculture, which favors the simplicity, convenience, and short-term success of herbicide use over more time-consuming strategies that can be burdensome to implement on farms (Shaner, 2000; Mueller et al., 2005; Johnson and Gibson, 2006; Sammons et al., 2007; Owen, 2008). Furthermore, increased reliance on glyphosate for weed control in glyphosate-resistant crops has reduced the price of other herbicides in the United States and has limited efforts to develop new herbicides (Shaner, 2000; Duke, 2005). Companies are increasingly focused on expanding the use of currently registered herbicides, which can be achieved by commercializing GE crops that are resistant to more than one herbicide (Duke, 2005; Green, 2007, 2009). Delaying the evolution of resistance to herbicides that are used with HR crops and minimizing other weed shifts are particularly important in this context because new herbicides may not be readily available to replace ones that become ineffective when resistance evolves. Therefore, farmers would benefit from focusing on more diverse, longer-term weed-management strategies to preserve the effectiveness of HR crops and to minimize the possibility of more expensive control tactics in the future.
ENVIRONMENTAL IMPACTS OF INSECT-RESISTANT CROPS
The adoption of Bt crops has changed insect-management strategies for most corn and cotton farmers in the United States. Those changes have implications for pest populations, soil conditions, and the management of insect pests in the future. The following section evaluates the impact of insect-resistant (IR) crop adoption on pest populations, on nontarget insects, and on soil quality. It also investigates resistance-management strategies and concerns related to the continued effectiveness of IR crops.
Levels of Insecticide Use
Insecticide Use in Corn
Insecticide use in corn (in pounds of active ingredient per acre) has steadily declined since 1997 as the adoption of Bt corn (which reached 50 percent of corn acres planted in 2007) has increased (Figure 2-7). Bt corn was introduced in the mid-1990s to control European corn borer (Ostrinia nubilalis). Because chemical control of European corn borer was not always profitable (and timely application was difficult) before the introduction of Bt corn, many farmers accepted yield losses rather than incur the expense and uncertainty of chemical control. For those farmers, the introduction of Bt corn resulted in yield gains rather than pesticide savings (Fernandez-Cornejo and Caswell, 2006). However, a new type of Bt corn introduced in 2003 to protect against corn rootworm (Diabrotica spp.), which was previously controlled with chemical insecticides and crop rotation, has provided substantial insecticide savings (Fernandez-Cornejo and Caswell, 2006).
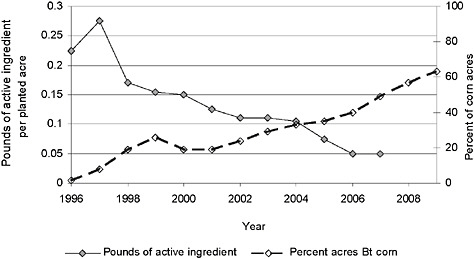
FIGURE 2-7 Pounds of active ingredient of insecticide applied per planted acre and percentage of acres of Bt corn, respectively.
NOTE: Seed-applied insecticide not included. Furthermore, the strong correlation between the rising percentage of Bt corn acres planted over time and the decrease in pounds of active ingredient per planted acre suggests but does not confirm causation between these variables.
SOURCE: USDA-NASS, 2001, 2003, 2005, 2007, 2009a, 2009b; Fernandez-Cornejo et al., 2009.
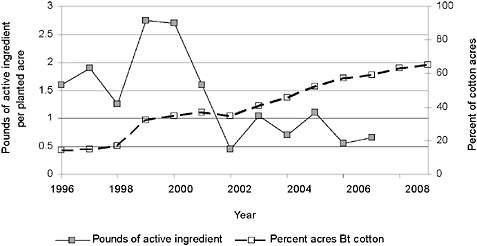
FIGURE 2-8 Pounds of active ingredient of insecticide applied per planted acre and percentage of acres of Bt cotton, respectively.
NOTE: The strong correlation between the rising percentage of Bt cotton acres planted over time and the decrease in pounds of active ingredient per planted acre suggests but does not confirm causation between these variables.
SOURCE: USDA-NASS, 2001, 2003, 2005, 2007, 2009a, 2009b; Fernandez-Cornejo et al., 2009.
Insecticide Use in Cotton
Cotton has the highest traditional use of insecticides per acre and the highest rate of adoption of Bt crops, reaching almost 60 percent in 2007, 12 years after Bt cotton was first commercialized (Figure 2-8). Insecticide use has fallen (in pounds of active ingredient per acre) over the same period, but fluctuations in total cotton insecticide applications have also been strongly affected by the boll weevil eradication program9 (Fernandez-Cornejo et al., 2009).
Regional Pest Reductions
Corn and cotton that produce Bt toxins can cause high mortality in insect pest populations in which Bt-resistance alleles are rare. For example, mortality in pink bollworm (Pectinophora gossypiella) and tobacco bud-worm (Heliothis virescens) on Bt cotton with Cry1Ac protein is virtually 100 percent throughout the growing season (Tabashnik et al., 2000; Showalter et al., 2009). However, mortality in the moths Helicoverpa armigera and Helicoverpa zea10 on Cry1Ac cotton is typically lower than 95 percent and declines during the growing season (Kennedy and Storer, 2000; Olsen et al., 2005; Tabashnik et al., 2008; Showalter et al., 2009). Crops that produce more than one Bt toxin generally cause higher mortality than crops that produce a single toxin although declines in mortality during the growing season may still be observed (Adamczyk et al., 2001; Bommireddy and Leonard, 2008; Mahon and Olsen, 2009; Showalter et al., 2009).
Because Bt crops can cause high pest mortality, it has been postulated that one effect of the widespread use of Bt crops is a reduction in some pest populations regionally (Kennedy et al., 1987; Alstad and Andow, 1995; Roush, 1997; Gould, 1998; Kennedy and Storer, 2000; Storer et al., 2003). According to that idea, an area-wide decline in pest abundance could occur because replacing non-Bt crop fields with Bt crop fields eliminates suitable habitats for the pests. If females lay eggs on Bt plants and on non-Bt host plants, laying eggs on Bt plants could substantially reduce the number of surviving offspring produced by females and cause a decline in pest density (Riggin-Bucci and Gould, 1997; Carrière et al., 2003; Shelton et al., 2008). Models have suggested that the suppression of pest populations is more likely as mortality induced by Bt crops increases, the abundance of Bt crops and female movement between patches of Bt and non-Bt plants increase, and the net reproductive rate in patches of non-Bt hosts decreases (Carrière et al., 2003). However, polyphagous pest species (those able to feed on multiple types of plants) often exploit crops sequentially during the growing season, tracking changes in host suitability (Kennedy and Storer, 2000). In some cropping systems, the feeding options of such pests might be limited to only a Bt crop for a few generations, when it is the only suitable resource available. Thus, Bt crops could affect pest population dynamics even when the crops are relatively rare (Kennedy et al., 1987; Wu et al., 2008).
Long-term monitoring of insect-pest density before and after commer-
cialization of Bt crops has provided evidence that deployment of Bt crops influences pest population dynamics regionally. Table 2-3 contains the results of pest-monitoring studies in the United States and China. Most of the studies covered a single region where spatially explicit data on the distribution of Bt crops were not available, but in one study of pink boll-worm population density in Arizona from 5 years before to 5 years after introduction of Bt cotton, the abundance of Bt and non-Bt cotton fields in 15 cotton-growing regions was quantified with geographical information system technology (Carrière et al., 2003). In regions with less than an average of 65 percent Bt cotton in the second 5-year period, the introduction of Bt cotton had no consistent effect on population density of pink boll-worm; in regions with more than 65 percent Bt cotton, the introduction of Bt cotton decreased pink bollworm population density, and the extent of the decline increased as the percentage of Bt cotton increased. Those data are consistent with modeling results and suggest that pest-population suppression occurs if the area of Bt crops exceeds a threshold percentage of Bt cotton (Carrière et al., 2003). Another recent study of European corn borer conducted in five major U.S. corn-producing states indicated that suppressive effects of Bt corn depended on the extent of adoption of the technology (Hutchison et al., 2007).
Storer et al. (2008) noted that producers, extension agents, and pesticide appliers reported less serious insect-pest control problems in non-Bt crops, such as soybean and vegetables, after the regional suppression of European corn borer and corn earworm (H. zea) by Bt corn in Maryland. As a comparison to the U.S. experience, a study conducted in six provinces of China from 1997 to 2006 documented a progressive decline in the population density of cotton bollworm (H. armigera) after the introduction of Bt cotton (Wu et al., 2008; Table 2-3). The significant suppression of cotton bollworm occurred not only in Bt and non-Bt cotton but in corn, peanut, soybean, and vegetables. Wu et al. (2008) proposed that the regional decline of cotton bollworm populations could reduce insecticide use in crops other than cotton. Nevertheless, the economic consequences of the regional suppression of pests by Bt crops have been investigated only for European corn borer in five U.S. Corn Belt states (Hutchison et al., 2007). It was estimated that regional declines in European corn borer population densities during the last 14 years in those states saved at least $3.9 billion for producers of non-Bt corn and $6.1 billion for producers of Bt and non-Bt corn combined. Further detailed spatially explicit studies of the association between the distribution of Bt crops and pest problems on different scales will be helpful in improving understanding of how the use of Bt crops can reduce pest abundances (Marvier et al., 2008).
The use of Bt crops sometimes changes pest-management practices enough to increase problems related to pests that are not killed by Bt
TABLE 2-3 Regional Effects of Deployment of Bt Crops on Population Dynamics of Major Pests of Corn and Cotton
Pest |
Location |
Regional Use of Bt Crops |
Number of Years Monitored |
Population Decline |
Reference |
Heliothis virescens |
Mississippi (Washington County) |
Up to 85% Bt cotton |
19 (1986–2005) |
Yes |
Adamczyk Jr. et al., 2001 |
Helicoverpa zea |
Mississippi (Washington County) |
Up to 85% Bt cotton |
19 (1986–2005) |
Yes |
Adamczyk Jr. et al., 2001 |
Heliothis virescens |
Louisiana (Bossier City) |
Unknown |
20 (1986–2005) |
Yes |
Micinski et al., 2008 |
Helicoverpa zea |
Louisiana (Bossier City) |
Unknown |
20 (1986–2005) |
No |
Micinski et al., 2008 |
Ostrinia nubilalis |
Maryland (Eastern Shore) |
Up to 60% Bt corn |
35 (1973–2007) |
Yes |
Storer et al., 2008 |
Helicoverpa zea |
Maryland (Eastern Shore) |
Up to 60% Bt corn |
35 (1973–2007) |
Yes |
Storer et al., 2008 |
Helicoverpa armigera |
China (Six provinces) |
Up to 10% Bt cotton |
10 (1997–2006) |
Yesa |
Wu et al., 2008 |
Ostrinia nubilalis |
Minnesota, Illinois, Iowa, Nebraska, Wisconsin |
Up to 75% Bt corn |
ca. 45 years (1943–2007)b |
Yes |
Hutchison et al., 2007 |
Pectinophora gossypiella |
Arizona (15 regions) |
0.01–87% Bt cotton |
10 (1992–2001) |
Yes |
Carrière et al., 2003 |
aStudy analyzed decline in density of H. armigera after commercialization of Bt cotton. bData analyzed for about 45 years in each state. Not all states were monitored each year in the period 1943–2007. |
toxins. For example, substantial reductions in the use of synthetic insecticides on Bt cotton favored outbreaks of mirids and leafhoppers in China (Wu et al., 2002; Men et al., 2005). Those pests had been well controlled by insecticides before the introduction of Bt cotton. Similarly, lower use of insecticides in Bt cotton probably contributed to the higher stink bug damage in cotton in some southern U.S. states although the regional increases in stink bug populations were probably influenced by other factors as well (Greene et al., 2001, 2006). Changes in pest-management practices in connection with Bt crops can also have favorable consequences for the control of some pests that are not killed by Bt toxins. For example, a reduction in insecticide use on Bt cotton was sometimes associated with greater predator abundance and better pest control in cotton aphid in the United States (see section “Natural Enemies”).
Reversal of Insect Resistance to Synthetic Insecticides
The deployment of Bt crops is known to promote a reversal of pest resistance to synthetic insecticides, but this has not yet been observed in the United States. In northern China, the reduction in use of insecticides on Bt cotton contributed to restoring cotton bollworm (H. armigera) susceptibility to some synthetic insecticides (Wu et al., 2005; Wu, 2007) although fitness costs associated with insecticide resistance likely helped to increase susceptibility. Similarly, resistance to pyrethroid insecticides declined considerably in tobacco budworm (H. virescens) after the introduction of Bt cotton in southern Tamaulipas, Mexico (Terán-Vargas et al., 2005). The renewed efficacy of insecticides provided more pest-management options to producers in those regions. However, such reversals in insecticide resistance do not always occur. For example, the planting of Bt cotton in Louisiana did not change the high levels of pyrethroid resistance in tobacco budworm (H. virescens) and cotton bollworm (H. zea) (Bagwell et al., 2001), and H. zea resistance to pyrethroids increased substantially after the planting of Bt cotton in several regions of Texas (Pietrantonio et al., 2007).
Effects on Nontarget Species
Bt toxins are considered acutely toxic to a relatively narrow array of invertebrate taxa when compared with broad-spectrum insecticides because toxicity through direct ingestion of a Bt toxin is typically restricted to insects in the same order as the target pest (Schnepf et al., 1998; Glare and O’Callaghan, 2000; van Frankenhuyzen and Nystrom, 2002; Mendelsohn et al., 2003). For example, the endotoxins Cry1Aa, Cry1Ab, and Cry1Ac kill mainly particular moths and butterfly species, while Cry3Aa and
Cry3Bb mainly kill particular beetle species. Furthermore, because Bt toxins are specific, they cause different mortality within targeted insect orders. For example, the cotton cultivar Bollgard I®, which produces the toxin Cry1Ac and targets lepidopteran pests, kills virtually 100 percent of pink bollworm and tobacco budworm (H. virescens), between 24–95 percent of cotton bollworms H. zea and H. armigera, and less than 4 percent of fall armyworm (Spodoptera frugiperda) and beet armyworm (Spodoptera exigua) (Showalter et al., 2009). Field studies have revealed relatively few adverse effects of Bt crops on arthropods that are not closely related to the target pests (Cattaneo et al., 2006; Romeis et al., 2006). In contrast, broad-spectrum insecticides, such as pyrethroids and organophosphates had consistent, adverse effects on a wide array of nontarget arthropods (Cattaneo et al., 2006; Romeis et al., 2006).
Although the high specificity of Bt crops for the control of target pests is consistent with integrated pest management, they may have effects on beneficial organisms. For example, the larvae of nontarget moths or butterflies in the landscape surrounding farms may be susceptible to Bt toxins that target pests in this group, but they would need to eat the Bt plant material to be affected. Bt corn byproducts that enter streams may affect aquatic insects in related taxa (Rosi-Marshall et al., 2007). The abundance of some natural enemies may decrease when their host or prey species are susceptible to Bt toxins and as a result become rare or nutritionally less suitable (Romeis et al., 2006).
Quantifying and predicting the effects of Bt crops on nontarget invertebrate species has been the subject of considerable work. As compiled by Marvier et al. (2007) and Naranjo (2009), research on the nontarget effects of Bt crops includes 135 laboratory studies of nine Bt crops and 22 Bt Cry proteins or protein combinations and 63 field studies of five Bt crops and 13 Bt proteins. In total, field and laboratory studies of at least 99 and 185 invertebrate species, respectively, have been conducted although not with equal effort. Most of the field studies have been of corn and cotton. Individual study results vary, so evidence-based generalizations are elusive in the absence of formal approaches. A review of recent syntheses provides an overview of the generalizations that have emerged thus far from those efforts.
For cotton and corn, whether Bt crop fields have more or fewer non-target invertebrates depends on whether one compares the Bt crop to a conventional counterpart that received insecticide treatments (Marvier et al., 2007; Wolfenbarger et al., 2008; Naranjo, 2009). Collectively, studies have indicated that a higher total abundance of arthropods occurred in Bt fields than in conventional fields sprayed with insecticides and a lower abundance than in conventional fields with no insecticide treatment (Marvier et al., 2007). For Bt corn, the magnitude of the effect also
depended on whether studies tested Bt176 (no longer registered for use) or the MON810 (commercially used) Bt events. Lower abundance of specific taxa was found in Bt fields than in unsprayed, non-Bt fields; the taxa in question included moths, butterflies, beetles, and true bugs on cotton and wasps on corn. Differences in the availability of prey or in survival may explain those results (Marvier et al., 2007).
Bt potato crop fields without insecticide use contained higher abundances of predators, natural enemies as a whole, and nontarget pests compared to conventional potato fields, whether or not insecticides were applied to the conventional fields (Wolfenbarger et al., 2008).
Natural Enemies
Maintenance of healthy populations of predators of crop pests is a desirable goal for ensuring long-term environmental sustainability of farms. Decreasing the numbers of predators, which in practice will be related to the overall biodiversity in an area and to on-farm pest control (Landis et al., 2008), would be undesirable. Even in systems where a single predator may suffice as a biocontrol agent, redundancy is an important tool for ensuring ecosystem services.
The few studies comparing biological control (by parasitism and predation rates) between Bt and conventional crops have suggested that control of nontarget pests on Bt crops was enhanced on cotton (Head et al., 2005) or similar on cotton (Naranjo, 2005) and corn (Pons and Starý, 2003; Naranjo, 2005) and that control of target pests on Bt crops was enhanced on cotton (Head et al., 2005), similar on cotton (Sisterson et al., 2004b; Naranjo, 2005) and corn (Orr and Landis, 1997; Sisterson et al., 2004b; Naranjo, 2005), or reduced on corn (Siegfried et al., 2001; Bourguet et al., 2002; Manachini, 2003; cited by Naranjo, 2009). Maintenance of biological control of nontarget pests in one study occurred in Bt cotton fields in spite of about a 20-percent reduction in the abundance of some common predators (Naranjo, 2005). When Bt crops have completely replaced insecticide-treated conventional crops, studies have consistently reported higher numbers of predators on cotton, corn, and potato. When Bt crops have replaced non–insecticide-treated conventional crops, results studies have consistently indicated slightly fewer predators on Bt cotton and no detectable difference on Bt corn (Wolfenbarger et al., 2008; Naranjo, 2009).
Field studies of parasitoids have overemphasized specialist species of the target pest of Bt corn, so generalizations to parasitoids as a group are premature. The studies have revealed a pattern similar to that of predators: fewer parasitoids in conventional corn fields sprayed with insecticides and no detectable difference between Bt corn fields and conventional corn fields not treated with insecticides. Laboratory studies
indicate that effects of Bt toxins on parasitoids depend on whether they are fed prey that are susceptible to Bt toxins (Zwahlen et al., 2000; Dutton et al., 2002; Schuler et al., 2003, 2004; Romeis et al., 2006). Syntheses of laboratory studies of 14 parasitoid species indicate a favorable or neutral effect on life-history traits when they were fed prey that had ingested a Bt toxin but were not affected by it (high-quality prey). Conversely, studies have shown longer development times, lower reproduction, and lower survival if the parasitoids were fed prey that had ingested a Bt toxin that was toxic to them (low-quality prey) (Naranjo, 2009).
The adoption of Bt cotton increases abundances of natural enemies and hence the potential for biological control when it completely replaces insecticide treatments. Moth larvae were responsible for a large fraction of cotton-insect losses before the adoption of Bt cotton, but cotton-insect losses caused by these larvae have become less important now that Bt cotton has been widely adopted. The five major insect pests of cotton in the United States in 2008 were lygus bugs (1 percent yield loss), boll-worms and budworms (0.76 percent yield loss), stink bugs (0.75 percent yield loss), thrips (0.52 percent yield loss), and cotton fleahoppers (0.23 percent yield loss) (Williams, 2009). Among those, only bollworms and budworms are controlled by Bt cotton, so the use of Bt cotton rarely eliminates all insecticide applications. Actual farm-level reductions in insecticide use for Bt cotton would probably increase the abundance of nontarget insects less consistently (e.g., Cattaneo et al., 2006; Sisterson et al., 2007) than what has been observed in experimental studies in which the use of Bt cotton completely replaced insecticide treatments.
Pollinators and Other Valued Insects
The honey bee (Apis mellifera) is one of the agricultural sector’s most important pollinators. Laboratory toxicity studies of honey bees have consistently found no evidence that Bt pollen or Bt proteins decrease honey-bee larval or adult survival (Duan et al., 2008) even at toxin concentrations well beyond what would be encountered in the field. There have been laboratory or field studies of few other species (Wolfenbarger et al., 2008; Naranjo, 2009); no consistent effect on development time (eight studies) or survival (20 studies) has been detected in laboratory tests, but effects varied widely among studies, particularly for development time (Naranjo, 2009). Laboratory studies collectively have indicated longer development time and lower survival of valued insect herbivores, a category that includes charismatic species (e.g., monarch butterfly larvae) and moths of economic importance (e.g., the silkworm) (Naranjo, 2009).
Summary of Nontarget Effects
The abundance of natural enemies on Bt crops can be greater than, the same, or lower than on non-Bt crops. The magnitude of the benefit depends on the extent to which a Bt crop substitutes for the use of insecticide treatments of non-Bt crops and on whether insecticides for other pests are used on the Bt crop. Honey-bee adults and larvae were not harmed by Bt pollen or Bt proteins, but too few pollinators have been studied to support generalizations about the group as a whole. As the sophistication of GE-crop varieties increases and the functional roles of arthropods become understood more fully, it should be possible to develop strategic pest-management systems that maintain high crop productivity while avoiding effects on nontarget moths, butterflies, and beetles.
Soil Quality
Overall, it appears that current Bt crops have no greater or lesser effect on soil quality than the crops that they have replaced. Many peer-reviewed studies have addressed the nontarget impacts of Bt crops on soil organisms. Specifically, studies have considered the effects of plant residues on the soil community because plants are the primary source of carbon in soils. If Bt toxins affect soil microorganisms, rates of decomposition and nutrient cycling may be altered. Studies have also focused on the consequences of Bt-containing root exudate. Root exudate influences the soil community, especially the community of distinct, specialized soil microorganisms associated with roots.
Most assessments of the effects of Bt insecticidal proteins on soil microorganisms and other organisms have found that these proteins do not substantially alter microbial populations and measured functions (Icoz and Stotzky, 2008). Over four years of continuous corn cultivation, Bt plant residues and root exudates had no consistent or persistent effect on a breadth of microorganisms or their enzymatic activity in the soil, but differences were detected according to plant species, variety, and age as well as other environmental factors (Icoz et al., 2008). With respect to macro-organisms, Lang et al. (2006) found no significant differences in earth-worm or springtail population density or biomass between soils with Bt and with non-Bt corn or between soils with corn treated and not treated with insecticide (baythroids) at five sites during 4 years of corn cultivation. Instead, the site and the sampling years had a greater influence on earthworm population density and biomass than the presence of the Cry protein. Those results corroborate other laboratory and field studies of the effect of Bt toxins on survival, growth, and reproduction of an array of soil invertebrates, including woodlice, springtails, and mites (Ahl Goy et al.,
1995; Saxena and Stotzky, 2001a; Zwahlen et al., 2003; Clark et al., 2006; Vercesi et al., 2006; Krogh et al., 2007). Similarly, Birch et al. (2007) detected transient and site-specific reductions in the biomass of oribatid mites and total microarthropods in fields under Bt and non-Bt corn. However, the differences between populations under different non-Bt corn varieties were often of the same magnitude as those between Bt and non-Bt corn; this led to the conclusion that the effects in the field were varietal effects and not due specifically to the Bt trait (Cortet et al., 2006).
When the effects of Bt toxins on nematodes were studied, season, soil tillage, soil type, crop type, and cultivar influenced nematode number to a greater extent than whether the corn was a Bt variety. Under field cultivation for Bt crops with the Cry3Bb1 protein and non-Bt crops, no effect was detected on the abundance of the nematode Caenorhabditis elegans on corn (Al-Deeb et al., 2003) or on the relative abundance of species of nematodes in the soil with eggplant (Manachini, 2003; cited by Icoz and Stotzky, 2008). When soils from Bt corn with the Cry1Ab protein and non-Bt corn in cultivation were compared, there were no effects on nematode communities and diversity (Manachini, 2003; cited by Icoz and Stotzky, 2008) or on the nematode Pratylenchus spp. (Lang et al., 2006). However, in experiments in cultivated Bt and non-Bt corn fields, adverse effects on growth and abundance of C. elegans were observed (Manachini, 2003; Lang et al., 2006; cited by Icoz and Stotzky, 2008), and a lower abundance of natural populations occurred transiently in Bt fields and consistently at one Bt site (Griffiths et al., 2005, 2006).
Similarly, the Cry1Ab insecticidal protein for European corn borer control had less effect on the bacterial community structure than other environmental factors (Baumgarte and Tebbe, 2005). In one study, a transient decrease occurred in the numbers of protozoa in soil with Bt corn under field conditions (Griffiths et al., 2005); otherwise, no toxic effects of the Cry proteins on protozoa have been observed (Donegan et al., 1995; Saxena and Stotzky, 2001a; Griffiths et al., 2005; Icoz and Stotzky, 2008). No changes in microbial activity and other assays (i.e., nitrogen mineralization potential, short-term nitrification, and soil respiration rate) occurred when soils cropped with corn that produced the Cry3Bb toxin for corn rootworm protection were compared with soils cropped with the nontransgenic isoline (Devare et al., 2004, 2007). Those studies have indicated that Bt and non-Bt crops have comparable effects on soil bacteria and protozoa.
Rates of residue decomposition and the associated accumulation of soil organic matter affect soil productivity and soil ecological functions; therefore, if residues of Bt corn differ from residues of non-Bt corn in decomposition rates, there might be long-term implications for soil quality and soil carbon sequestration. Reduced decomposition rates might increase the time that Bt toxins remain in the environment. Chemical
bonds in lignins are more resistant to microbial decomposition than other chemical bonds in plant cells. Some studies have demonstrated higher lignin content in Bt corn hybrids compared to their respective isolines (or near-isolines) (Saxena and Stotzky, 2001b; Poerschmann et al., 2005), but other studies have found no differences (Jung and Sheaffer, 2004). Decomposition rates have more importance for soil quality than relative lignin contact. In some laboratory research, plant residue of Bt hybrids decomposed at a lower rate in soil than residue of non-Bt hybrids (Flores et al., 2005), but field studies have not detected differences in decomposition rates (Lehman et al., 2008). Similarly, Hopkins and Gregorich (2003) reported no differences in carbon dioxide production from Bt and non-Bt corn in soil over a 43-day incubation period. No differences in mass losses in the field were detected between Bt-glyphosate–resistant and glyphosate-resistant cotton lines, indicating that there were no differences in the rate of decomposition or change in nutrient content in the litter over the 20-week experiment (Lachnicht et al., 2004). When the whole soil organism community was allowed to access the residue, the decomposition of Bt and non-Bt residue was similar (Zwahlen et al., 2007). Tarkalson. Tarkalson et al. (2008) also reported no differences in residue decomposition rates or in mass of total carbon remaining over time between Bt and non-Bt corn hybrids observed in a field study although the study did detect differences in rates of decomposition for leaf, stalk, and cob plant parts. Finally, after seven years of continuous corn cultivation, no differences between Bt and non-Bt corn treatments were detected in total carbon or nitrogen in soil, indicating that plant decomposition rates were similar (Kravchenko et al., 2009). On the basis of those studies, the plant residue from Bt and non-Bt corn hybrids decomposed at similar rates and would have similar effects on soil quality and on potential carbon sequestration.
Evolution and Management of Insect Resistance
Evolution of Resistance
Insects can adapt to toxins and other tactics used to control them (Palumbi, 2001; Onstad, 2008). When Bt crops were first considered for commercial introduction, EPA recognized their potential to reduce human and environmental exposure to broad-spectrum insecticides, increase growers’ ability to manage pests and improve crop quality, and increase profits at the farm and industry levels (Berwald et al., 2006; Matten et al., 2008). Those benefits had already been demonstrated by sprayed Bt insecticides that are critical pest-management tools for many fruit and vegetable crops in the United States (Walker et al., 2003a). As the regulatory agency overseeing the introduction of biological pesticides, EPA concluded that the potential
for rapid evolution of insect resistance to Bt toxins produced by GE crops was a threat to the benefits provided by Bt crops and to the efficacy of Bt sprays in organic and conventional production systems (Matten et al., 2008; Thompson et al., 2008). Accordingly, it mandated the use of a refuge strategy (described later in this chapter) to delay the evolution of resistance in major insect pests controlled by Bt corn and cotton (US-EPA, 2008a).
Extensive monitoring of 11 major lepidopteran pests of corn and cotton over the last 14 years has revealed that some populations of one moth species, cotton bollworm (Helicoverpa zea), evolved resistance to the Bt toxins Cry1Ac and Cry2Ab found in some cotton cultivars in the United States (Tabashnik and Carrière, 2008; Tabashnik et al., 2008, 2009a). In addition, some populations of fall armyworm evolved resistance to Cry1F corn in Puerto Rico (Matten et al., 2008), and some populations of corn stem borer (Busseola fusca) evolved resistance to Cry1Ab corn in South Africa (van Rensburg, 2007; Kruger et al., 2009).
That resistance has evolved in only three pest species in the last 14 years suggests that the refuge strategy has successfully delayed the evolution of resistance to Bt toxins (Tabashnik et al., 2008, 2009a). Comparisons between pests that have and have not evolved resistance to Bt crops suggest that recessive inheritance of resistance and abundant refuges of non-Bt host plants are two key factors that delay the evolution of resistance (Tabashnik et al., 2008, 2009a). In accordance with these findings, EPA demands that GE seed companies require producers to plant refuges to delay the evolution of resistance where such refuges are deemed necessary and develop compliance assurance programs (Thompson et al., 2008). The promotion of precise resistance-management guidelines by the industry has undoubtedly contributed to increasing the use of refuges for managing the evolution of resistance to Bt crops in the United States. In some regions, compliance to the mandated refuge strategies has been high since the introduction of Bt crops (Carrière et al., 2005). However, levels of compliance have substantially and regularly declined in other parts of the country, possibly because the use of Bt crops has increased globally and producers can no longer rely on non-Bt users to provide refuges for their farms (Jaffe, 2009).
Although the theory, resistance-monitoring data, and experimental work conducted in the laboratory suggest the refuge strategy has been useful, detailed field experiments are still needed to demonstrate how the refuge strategy can delay the evolution of resistance to Bt crops. There is usually a delay between the introduction of a novel pesticide and the rapid rise in the number of species that have evolved resistance to it (Georghiou, 1986). That is illustrated in a comparison of the cumulative number of cotton pests that evolved resistance to Bt toxins in crops and to
the insecticide dichlorodiphenyltrichloroethane (DDT) after the introduction of these pest-management tools in the United States (Figure 2-9).
After commercialization of Bt cotton in 1996, its use increased rapidly in the United States. Similarly, the use of DDT in cotton increased rapidly after it became widely commercially available in 1946. For example, 90 percent of agricultural DDT applications in the United States targeted cotton pests in 1962 (Walker et al., 2003b). Similar to Bt cotton that produces high concentrations of Bt toxins over much of the growing season, DDT was applied repeatedly in cotton and retained toxicity for extended periods (US-EPA, 2000). The recessive mutations kdr and super-kdr confer recessive resistance to DDT in many agricultural pests (Davies et al., 2007; APRD, 2009); this is similar to the inheritance of resistance to Bt toxins in cotton, which is often recessive (Tabashnik et al., 2008).
With respect to the evolution of resistance, Bt cotton and DDT differ in at least two important ways. First, DDT kills a wide array of insects regardless of their feeding habits whereas Bt cotton kills only some lepidopteran pests that feed on the cotton. Second, no refuge strategy was mandated to manage the evolution of insect resistance to DDT. Those differences suggest that the evolution of DDT resistance in cotton pests should have been more rapid than the evolution of resistance to Bt cotton. However, the cumulative number of cotton pests that evolved resistance to Bt cotton and the number that evolved resistance to DDT after their introduction in the United States have been strikingly similar (Figure 2-9). That comparison indicates that it may still be too soon to claim that the refuge strategy has substantially delayed the accumulation of pests resistant to Bt. While seed companies are in a better position to commercialize more efficient Bt cultivars for delaying the evolution of resistance (see below), the possibility remains that the accumulation of resistant pests could accelerate. Thus, complacency in the implementation of resistance-management strategies is not warranted (Hurley and Mitchell, 2008; Jaffe, 2009; Tabashnik et al., 2009a).
Principles of Population Genetics Underlying the Refuge Strategy
Population-genetic models and empirical data on factors that affect the evolution of insect resistance to Bt crops have been central in the development of the refuge strategy. Models generally assume that resistance to a toxin produced by Bt crops is conferred by mutations at a single locus (gene location) (Gould, 1998; Tabashnik and Carrière, 2008). That is a reasonable assumption because resistance to the intense selection imposed by Bt crops and insecticides is likely to involve genes that have major effects (Carrière and Roff, 1995; McKenzie, 1996). Furthermore, most of the observed cases of evolved resistance to Bt crops have involved

FIGURE 2-9 Cumulative number of cotton pests evolving resistance to Bt cotton and DDT in the years after these management tools became widely used in the United States.
SOURCE: APRD, 2009.
mutations at a single locus (Gahan et al., 2001; Morin et al., 2003; Yang et al., 2007; Pereira et al., 2008). For simplicity, models assume the presence of one allele that confers susceptibility and one allele that confers resistance even if more than one allele at a single locus can confer resistance to Bt crops (Morin et al., 2003; Yang et al., 2007).
The refuge strategy relies on two basic principles. The first principle is that the dominance of resistance11 is reduced by increasing the dose of Bt toxins (Gould, 1998; Tabashnik et al., 2004). When the concentration of a Bt toxin in a plant is low, the resistance trait in the insect population is nonrecessive, but when it is high, the resistance trait in the insect population becomes recessive, and resistance becomes rarer. Accordingly, resistance to commercialized GE crops that produce high concentrations of Bt toxins is recessive in many, but not all, target pests (Tabashnik et al., 2008). The refuge strategy requires the presence of refuges of non-GE host plants in or near Bt crop fields (US-EPA, 2008a, 2008b). For refuges to be effective, the susceptible insects produced in refuges must be in sufficient
numbers and mate with the rare resistant pests that survive on GE crops. With effective refuges and recessive resistance, most hybrid offspring produced by resistant pests that survive on Bt crops are killed when they feed on GE crops. That reduces the heritability of resistance (the degree of genetic similarity between resistant parents that survive on Bt crops and their offspring) and delays its evolution (Gould, 1998; Sisterson et al., 2004a; Tabashnik and Carrière, 2008).
The second principle underlying the refuge strategy is that the evolution of resistance can be delayed or prevented by reducing the selective differential between individuals with and without resistance alleles (Gould, 1998; Carrière and Tabashnik, 2001; Andow and Ives, 2002; Tabashnik et al., 2005; Crowder and Carrière, 2009). The selective differential between resistant and susceptible individuals can be affected by crop-management practices, such as increasing refuge size that increases relative fitness of susceptible individuals (Mitchell and Onstad, 2005; Onstad, 2008). In fields of Bt crops, where resistant individuals are more abundant than susceptible individuals, the selective differential between resistant and susceptible individuals can be reduced by such crop-management practices as pheromone mating disruption and the elimination of crop residues that contain insects (Andow and Ives, 2002; Carrière et al., 2004b).
The selective differential between resistant and susceptible individuals can also be affected by pest biology and genetics. Fitness costs associated with resistance to Bt toxins occur in environments that lack Bt toxins if individuals with one or more resistance alleles have lower fitness than individuals without such alleles (Gassmann et al., 2009). Fitness costs of Bt resistance are found in many species and select against resistance in environments where Bt toxins are absent; this selection counterbalances selection that favors an increase in resistance in fields of Bt crops (Gassmann et al., 2009). Fitness costs expressed in heterozygous individuals are non-recessive; costs expressed only in homozygous resistant individuals are recessive. Nonrecessive fitness costs can delay the evolution of resistance more effectively than recessive fitness costs because alleles that confer resistance to Bt crops are often rare (Gould et al., 1997; Andow et al., 2000; Tabashnik et al., 2006; Mahon et al., 2007), so most resistance alleles in pests targeted by Bt crops are carried by heterozygous individuals. With nonrecessive fitness costs, the fitness of resistant heterozygous individuals is lower than the fitness of susceptible individuals in refuges, and such costs can strongly select for a decline in resistance despite the fact that selection favors resistant individuals on Bt crops (Gassmann et al., 2009). In other words, recessive costs that influence only the rare homozygous resistant individuals are less effective in delaying resistance than non-recessive costs that influence the more abundant resistant heterozygous individuals.
Incomplete resistance occurs when the fitness of resistant individuals is lower on Bt cultivars than on corresponding non-Bt cultivars (Carrière and Tabashnik, 2001). It occurs because the individuals that do survive on Bt crops are nevertheless affected adversely by Bt toxins (for example, larvae take a long time to develop on the Bt crop, and the resulting moths are smaller and less fecund). Incomplete resistance is found in many species and contributes to delaying the evolution of resistance by reducing the selective differential between resistant and susceptible individuals (Carrière and Tabashnik, 2001; Tabashnik et al., 2005; Crowder and Carrière, 2009).
The Pyramid Strategy
The first IR crops produced a single Bt toxin. More recently, the “pyramid” strategy has used GE crops that produce two distinct Bt toxins for delaying pest resistance. The pyramid strategy is based on the principle that insects are killed on two-toxin plants as long as they have a susceptibility allele at a resistance locus—a phenomenon called redundant killing (Gould, 1998; Roush, 1998). As resistance alleles are generally rare, the only genotype that has high survival on a cultivar that produces two or more Bt toxins is expected to be extremely rare. Accordingly, the refuge strategy is considered more effective in reducing the heritability of resistance when crops produce more than one Bt toxin than when they produce a single Bt toxin (Gould, 1998; Roush, 1998; Gould et al., 2006). Models suggest that the pyramid strategy is most effective when the majority of susceptible pests are killed by the GE crop, resistance to each Bt toxin is recessive, fitness costs and refuges are present, and selection with one Bt toxin does not cause cross-resistance to another (Gould, 1998; Zhao et al., 2005; Gould et al., 2006). Cross-resistance to Bt occurs when a genetically based decrease in susceptibility to one toxin decreases susceptibility to other toxins.
Changes in Refuge Strategy in the United States
In a process that aims to use scientific knowledge to balance economic and environmental considerations, refuge strategies for Bt corn and cotton mandated by EPA have been improved since the commercialization of these GE crops in 1996. EPA specifies the area, configuration, and types of refuges to be used with specific Bt crops. Changes in refuge requirements have been based on input from academe, farmers, and industry. Some of the changes have made refuge requirements more stringent, while others have eliminated refuge requirements. For example, refuge distance requirements for the use of Bt cotton against pink bollworm
were unspecified from 1996 to 2000, unless the percentage of Bt cotton in a county exceeded 75 percent in the previous year (US-EPA, 1998; Carrière et al., 2001). However, on the basis of the principle that refuges must be near Bt crops to promote the desired mating between susceptible and resistant insects, new regulations enacted in 2001 limited the distance between refuges and Bt cotton regardless of the percentage of Bt cotton in the previous year (Tabashnik et al., 1999; Carrière et al., 2001; US-EPA, 2001; Carrière et al., 2004a). Since 2006, in response to a proposal from cotton growers to eradicate pink bollworm in Arizona, EPA has allowed use of mass release of sterile pink bollworm moths as an alternative to non-Bt cotton refuges (US-EPA, 2006a).
In another example, in response to a proposal from Monsanto, the refuge requirement of non-Bt cotton cultivars was abolished from Texas to the Mid-Atlantic to manage resistance of tobacco budworm (Heliothis virescens) and cotton bollworm (Helicoverpa zea) to Monsanto’s pyramided Bt cotton cultivar that produces the toxins Cry1Ac and Cry2Ab (US-EPA, 2007) and subsequently to a cultivar from Dow AgroSciences producing Cry1Ac and Cry1F. The proposal included new data and modeling results that indicated that weeds and non-Bt crops other than cotton might provide sufficient refuges to delay Bt resistance in the two mobile, polyphagous pests (US-EPA, 2006b). The 2007 change by EPA meant that refuges of non-Bt cotton are no longer required for millions of acres of the Monsanto cotton cultivar grown in large areas of the United States. Assuming that no other factors changed (e.g., the technology fee), that action would improve the net benefits to farmers growing the GE cotton and increase its adoption (Luna V. et al., 2001; Matus-Cádiz et al., 2004) at least in the short term. However, it is noteworthy that decreased susceptibility to both Cry1Ac and Cry2Ab in cotton bollworm (H. zea) has indicated that this pest is evolving resistance to cotton producing those toxins in the United States (Tabashnik et al., 2008, 2009b).
It appears likely that most Bt crops commercialized in the future by biotechnology companies will produce two or more Bt toxins for the control of individual insect pest species (Bravo and Soberón, 2008; Matten et al., 2008). That could improve the durability of Bt crops if few other major insect pests targeted by Bt crops evolve resistance before the replacement of one-toxin crops by pyramids and if populations of pests that are resistant to single-toxin Bt crops remain rare. EPA promotes the replacement of one-toxin Bt cultivars with two-toxin Bt cultivars on the basis of recognition that the evolution of resistance is more effectively delayed with a pyramid strategy than with one-toxin crops (Matten et al., 2008). Another incentive to eliminating the use of one-toxin Bt cultivars when two-toxin Bt cultivars are introduced is that results from simulation models and small-scale laboratory experiments indicate that the evolution of resis-
tance to two-toxin cultivars is accelerated when plants that produce two Bt toxins are grown near plants that produce just one toxin (Roush, 1998; Gould, 2003; Zhao et al., 2005).
So far, the complete replacement of one-toxin with two-toxin Bt cultivars has occurred only in Australia (Baker et al., 2008). The Transgenic and Insecticide Management Strategy committee, which comprises growers, consultants, researchers, seed companies, and chemical industry, has overseen the development, implementation, and evaluation of resistance-management strategies for Bt cotton in Australia (Fitt, 2003). Cotton that produces the Bt toxin Cry1Ac for the control of cotton boll-worm (Helicoverpa armigera) was replaced by cotton producing Cry1Ac and Cry2Ab in 2004. That allowed producers to reduce the area of refuges from 70 percent with Cry1Ac cotton to as low as 5 percent with Cry1Ac and Cry2Ab cotton (Baker et al., 2008). The exclusive use of a pyramid strategy for managing the evolution of Bt resistance in insect pests might allow producers to use more Bt crops while maintaining efficient resistance management (Mahon et al., 2007; Baker et al., 2008). However, an allele conferring high levels of resistance to Cry2Ab has been found in relatively high frequency (0.0033) in field populations of cotton bollworm (H. armigera), and individuals homozygous for this allele can survive on mature cotton producing the toxins Cry1Ac and Cry2Ab (Mahon et al., 2007; Mahon and Olsen, 2009). This indicates that a key assumption of the pyramid strategy is not met (i.e., redundant killing), and thus that caution should be used to manage the evolution of cotton bollworm resistance to Cry1Ac and Cry2Ab cotton in Australia.
Agricultural and Environmental Impacts of Insect Resistance to Bt Crops
The refuge strategy was mandated in the United States not only to slow the evolution of resistance to Bt cultivars but also to protect the effectiveness of Bt sprays. Susceptibility to sprays with many Bt toxins in pests that evolve resistance to single-toxin Bt crops will depend on several factors, including the level of resistance, the variety and concentration of the toxins in the sprays, and the extent of cross-resistance to different toxins. If a spray contains one or more toxins to which the pest has evolved resistance or cross-resistance, susceptibility to the spray could be decreased (Tabashnik et al., 1993; Moar et al., 1995). Nonetheless, sprays containing one or more toxins that kill pests that are resistant to other toxins can be useful against such pests (Tabashnik et al., 1993; Liu et al., 1996; Akhurst et al., 2003; Wang et al., 2007).
Along with other insecticidal compounds with different modes of action, many sprayed Bt insecticides commonly used in the United States contain at least two Cry toxins that differ substantially from each other
in amino acid sequence and that bind to different target sites in the larval midgut (Schnepf et al., 1998; Ferré and Van Rie, 2002; Crickmore et al., 2009). The few pests that have evolved resistance to Bt crops could remain susceptible to sprayed Bt insecticides that contain many Bt toxins, as long as the evolution of resistance to the toxins in Bt crops does not involve strong cross-resistance to all the toxins in Bt sprays. Cross-resistance between Bt toxins that differ substantially in amino acid sequence is usually weak or nil, but exceptions occur in important pests that are targeted by Bt crops, including cotton bollworm (H. zea) (Hernández-Martínez et al., 2009; Tabashnik et al., 2009b). However, the possibility of cross-resistance in corn stem borer and fall armyworm has not been investigated extensively. The agricultural and environmental impacts of cross-resistance between single-toxin Bt crops and multitoxin Bt sprays will also depend on the extent to which the two approaches are used to control a given pest and on pest movement between Bt crops and areas where Bt sprays are used.
EPA requires remedial action plans to address cases of resistance, which can involve cessation of use of a particular Bt cultivar in a specific area (US-EPA, 1998; Carrière et al., 2001; US-EPA, 2001). Sales of corn that produce the Bt toxin Cry1F were suspended voluntarily in Puerto Rico after the evolution of resistance to Cry1F in fall armyworm (Matten et al., 2008). In the absence of published information on the distribution of resistance and on the presence of cross-resistance and fitness costs, it is not possible to assess whether the evolution of resistance to Cry1F in fall armyworm threatens the sustainability of other Bt crops or sprayed Bt insecticides in Puerto Rico. Furthermore, the economic and environmental consequences of Bt resistance are difficult to assess because little information is available on the profitability of Cry1F corn in Puerto Rico and on how withdrawal of Cry1F corn in Puerto Rico has affected insecticide use.
In contrast, the evolution of resistance of cotton bollworm (H. zea) to Cry1Ac cotton in the United States did not have serious agronomic, economic, or environmental consequences. That is because resistance did not affect many bollworm populations, Cry1Ac cotton still provides some control of Cry1Ac-resistant insects, synthetic insecticides were used in conjunction with Cry1Ac cotton from the onset to control bollworm, and the widespread use of cotton that produces both Cry1Ac and Cry2Ab in the states where resistance occurred provided effective control of insects that were resistant to Cry1Ac (Tabashnik et al., 2008). Data on increased bollworm survival on cotton plants that produce Cry1Ac and Cry2Ab in the field or on the consequences of field-evolved resistance to Cry2Ab are lacking (Tabashnik et al., 2009b). Although there is strong evidence of resistance to Cry1Ac in some populations of bollworm in the Southeast (Tabashnik and Carrière, 2009; Tabashnik et al., 2009a), a subset of the data
has been contested by some scientists (Moar et al., 2008), and EPA has not commented on the situation.
GENE FLOW AND GENETICALLY ENGINEERED CROPS
This section presents an overview of the potential of gene flow to weedy relatives for crops for which GE varieties have been developed (though not all of these varieties have been commercialized). The movement of herbicide resistance into weedy relatives present on farm fields can influence farmers’ weed-management strategies. Gene flow between GE and non-GE crops could accelerate the evolution of pest resistance to Bt crops, if many Bt plants are routinely present in refuges of non-Bt crops (Heuberger et al., 2009; Krupke et al., 2009). The following section specifically considers factors that affect gene flow via cross-pollination within crops, on which the coexistence of GE and non-GE crops depends. Chapter 3 addresses other sources of gene flow, such as co-mingling of seed and germination of volunteer seeds left behind, and the economic consequences of gene flow between GE and non-GE varieties.
Gene Flow Between Genetically Engineered and Non-Genetically Engineered Crops
The potential for cross-pollination between GE and non-GE crops depends on the plant species (Ellstrand, 2003a). In particular, the reproductive strategy of a crop determines the degree of gene flow between GE and non-GE crops; open-pollinated crops (such as corn) have the greatest probability of cross-pollination between GE and non-GE cultivars. Even in self-pollinated plants, out-crossing occurs occasionally, the rate depending on the particular species and environment. In soybean, for example, out-crossing is occasional (Palmer et al., 2001; Abud et al., 2004, 2007). In contrast, corn is freely out-crossing, so the cross-pollination of non-GE cultivars with pollen from GE varieties depends on the distance from the source and other factors. Models of pollen dispersal in corn and the consequent gene flow may have low precision, particularly in light of the small amounts of pollen that move more than 800 ft and the substantial impacts of climate (Ashton et al., 2000).
Among the factors that control gene flow between populations of wind-pollinated plants are distance from the pollen source and pollen shed density, time required for pollen movement, wind speed and direction, air temperature, and relative humidity (Luna V. et al., 2001; Westgate et al., 2003). Pollen viability declines quickly with desiccation. Even if pollen is dispersed over great distances, it may, if dried out, not be viable. Similarly, the occurrence of GE pollen in a non-GE corn field does not
necessarily mean that pollination will occur (Feil and Schmid, 2002). Nonetheless, the distances needed to prevent any cross-pollination in corn or other open-pollinated crops are so great that they are not practical in current commercial agricultural systems (Luna V. et al., 2001; Matus-Cádiz et al., 2004).
Insect-mediated cross-pollination between GE and non-GE crops occurs in those species for which insects typically are the agents of transfer of pollen between individuals (Van Deynze et al., 2005; Llewellyn et al., 2007). Canola and cotton are modally out-crossing as a result of pollinator activity, whereas soybean is usually self-pollinated but is visited by insects seeking its pollen (Ahrent and Caviness, 1994; Walklate et al., 2004; Heuberger and Carrière, 2009). In a recent study that monitored cross-pollination of seed-production fields of non-Bt cotton (some HR, some not) by Bt cotton, both the density of flower-foraging honey bees in seed production fields and the area of Bt cotton at a distance of 2,460 ft from the non-Bt cotton fields affected cross-pollination (Heuberger and Carrière, 2009). It had been documented that most cross-pollination in cotton occurs over distances of less than 160 ft (McGregor, 1976; Free, 1993; Xanthopoulos and Kechagia, 2000; Zhang et al., 2005). Nevertheless, foraging honey bees can easily travel two miles or more (Beekman and Ratnieks, 2000); this suggests that the 2,460-ft radius at which pollen from Bt cotton influenced out-crossing of non-Bt cotton resulted from movement of foraging honey bees from Bt to non-Bt cotton fields. Accordingly, the results of Heuberger and Carrière (2009) indicate that small-scale gene-flow studies may miss occasional long-distance cross-pollination between GE and non-GE insect-pollinated crops.
As the adventitious presence of GE traits is widespread in the seed supply of non-GE crops, gene flow between non-GE and GE crops may commonly involve cross-pollination by plants from the same field (i.e., adventitious plants). Heuberger and Carrière (2009) found adventitious Bt cotton plants in 67 percent of seed-production fields of non-Bt cotton. They demonstrated that adventitious Bt plants resulted both from human error (inadvertent planting of Bt cotton in non-Bt cotton fields) and from contamination in seed bags. After accounting for the effect of the area of Bt cotton surrounding a seed-production field and the abundance of foraging honey bees, the density of adventitious Bt plants was positively associated with out-crossing rates in seed-production fields. Most models of pollen transfer between crop varieties have not considered the adventitious presence of GE plants in non-GE fields and may therefore fall short of making accurate predictions of the abundance of GE traits in supposedly non-GE plants.
Canola is not a major crop in the United States, but substantial acreage is planted in North Dakota, which accounted for more than 87 percent
of U.S. canola planted in 2009 (USDA-NASS, 2009b). Gene flow between GE and non-GE canola is well documented in the large canola-growing region of western Canada; it can be facilitated by the transient populations of canola established each year outside agricultural fields (Knispel et al., 2008). Genetically engineered HR canola cultivars are the dominant type, and in 2006, glyphosate-resistant and glufosinate-resistant canola cultivars accounted 65 percent and 32 percent, respectively, of U.S. canola acres planted (Howatt, personal communication). Given that there are two GE herbicide-resistance traits (glyphosate and glufosinate) and a non-GE imidazolinone-resistant trait, introgression of these traits can result in multiple-herbicide resistance in a single plant (Knispel et al., 2008). The occurrence of multiple-herbicide–resistant volunteer canola increases the difficulties of management (Beckie et al., 2004; Beckie, 2006; Beckie et al., 2006). In addition to the problem of deploying special management techniques for HR weeds, adventitious presence of a GE trait in a non-GE field of canola has economic consequences.
Alfalfa is an important crop in the United States and is widely cultivated over a broad geographic range (USDA-NASS, 2008). GE glyphosate-resistant alfalfa was commercialized in 2005, and about 198,000 acres were planted in 2006 (Weise, 2007). However, in 2007, it once again became a regulated item, a decision that was upheld by the Court of Appeals in 2008. USDA Animal and Plant Health Inspection Service (APHIS) was ordered to conduct an environmental impact statement (EIS) because of “the significant threat of gene flow and the development of Roundup-resistant weeds that requires further study and analysis in an EIS” (Geertson Farms v. Johanns, 2009).12 APHIS released the draft EIS for public comment in December 2009.
Sugar beet (Beta vulgaris) cultivars with the GE trait that confer resistance to glyphosate have been commercialized and were widely adopted by growers in the United States. However, in September 2009, the Northern California District Court ruled that USDA violated the National Environmental Protection Act when it deregulated HR sugar beets, and USDA is required by the court to prepare an environmental impact statement to adequately consider the impacts of GE sugar beets on other sugar beet growers as well as farmers growing table beets and swiss chard, two crops with which sugar beets may cross pollinate (Center for Food Safety v. Vilsack, 2009).
Gene Flow Between Genetically Engineered Crops and Related Weed Species
In locations where crop varieties occur with wild or weedy populations of the same or closely related species, interbreeding between a crop and its relatives may lead to exchange of genes between the cultivars or species involved. Such hybridization is common in plants generally and is a key process for the evolution of new plant species. When gene flow occurs between crops and their wild relatives, an agronomic characteristic may move into the wild populations. Environmental sustainability on farms might be affected by the consequences of such gene flow between crops and wild relatives if the gene flow reduces genetic diversity available for crop improvement. However, only a few crops (sunflower, pecan, blueberry, and some squashes) were domesticated within the borders of the United States, so most crops planted on U.S. farms do not pose a risk to the conservation of genetic diversity in related native species and landraces. When crop species coexist with weedy relatives, gene flow might result in a weed-management issue and any accompanying economic and environmental effects. At least 15 crop species have been documented to hybridize with weedy relatives in the United States (Keeler et al., 1996). For HR traits, hybridization is a mechanism by which herbicide resistance might evolve in related weeds if HR crops are able to interbreed with related weedy species occurring in the same location (see the canola example in “Developing Weed-Management Strategies in Herbicide-Resistant Crops” earlier in this chapter).
In the United States for corn and soybean, the most common GE crops grown, no genetically compatible relatives or weedy strains exist; therefore, movement of GE traits into related weed species is not an issue. Wild populations of cotton (Gossypium hirsutum) exist along the Gulf Coast and a wild relative (Gossypium tomentosum) is in Hawaii. Gene flow is unlikely because the United States prohibits the commercial sale of Bt cotton in those areas. In Hawaii, test varieties and nursery stock can be produced, but with restrictions to minimize gene flow (e.g., US-EPA, 2005). In contrast, the use of HR crops in the same areas is apparently not more restricted than in the rest of the United States (USDA-APHIS, 2008).
Hybridization between the allotetraploid canola (Brassica napus) and one of its diploid weedy parents, turnip mustard (Brassica rapa) are extensive, and the hybrids are usually about 60 percent pollen fertile (Warwick et al., 2003; Légère, 2005; Simard et al., 2006), thus facilitating the spread of a GE trait into the weeds. GE herbicide-resistant traits have been reported to persist in populations of turnip mustard as they have in several other species of weeds (Warwick et al., 2003; Owen and Zelaya, 2005; York et al., 2005; Warwick et al., 2008). In addition, hybridization is possible between
canola and species of a few related genera of mustards, some of them weedy (Warwick et al., 2000; FitzJohn et al., 2007) and occurs spontaneously when they are grown together in an experimental garden. For these reasons, canola has been designated as a moderate-risk crop with regard to the potential for gene flow to its weedy relatives (Stewart et al., 2003), and farm-level effects may occur in canola-growing regions as discussed earlier. The extent to which they have economic impacts and affect environmental sustainability will depend on how the weeds are managed.
Gene flow has been demonstrated from sugar beet to near-relative weeds, B. macrocarpa and B. vulgaris subsp. maritima (Andersen et al., 2005). Thus, the introgression of HR traits from GE sugar beets to weedy beets (B. vulgaris) and sea beets (B. vulgaris subsp. maritima) should be considered a moderate risk (Stewart et al., 2003), but the consequences of gene flow would occur on a very small spatial scale in the United States. Co-occurrence of those species with sugar beet cultivation was limited to two California counties (Kern and Imperial) in 2006 (Calflora, 2009). Weedy beets are sporadic and local in the United States and not considered a major problem, as they are in Europe, where the species is native.
Transgenic virus-resistant squash has been available commercially since 1995 and was estimated to have been planted on an average of 12 percent of the 58,400 acres in squash production in 2005 (Quemada et al., 2008). Wild populations of Cucurbita pepo occur in south and central regions of the United States (Cowan and Smith, 1993) and can be an agricultural weed (Oliver et al., 1983). Gene flow between conventional cultivars of domesticated C. pepo and its wild populations is known to occur (Kirkpatrick and Wilson, 1988; Decker-Walters et al., 2009). Data are not available yet on the extent to which transgenes may exist in wild populations. As of 2005, the opportunity for gene flow across a large spatial scale appeared low because the majority of transgenic squash cultivation occurs outside the range of the wild populations of C. pepo (Quemada et al., 2008). The consequences of any gene flow to wild populations depend on virus incidence and the expression of the transgene in the wild populations (Spencer and Snow, 2001; Fuchs et al., 2004a, 2004b; Laughlin et al., 2009; Sasu et al., 2009)
Concerns about the consequences of a transfer of GE traits to wild or weedy populations and how to effectively mitigate those consequences have delayed the release of GE sunflower (Helianthus annuus, a species that was domesticated in the United States), creeping bentgrass (Agrostis stolonifera, a popular turf grass introduced from Europe), and rice (Oryza sativa, a species native to Asia with related, intercompatible weeds introduced into some rice fields with the crop). For sunflower, the potential for transgene movement to weedy relatives is quite high and thus the consequences of gene flow on weed management presents an environ-
mental concern about the commercial development of transgenic sunflower (Snow and Palma, 1997; Snow, 2002; Ellstrand, 2003b; Stewart et al., 2003). Wild sunflowers are weeds in row-crop fields, including corn, soybean, domesticated sunflower, wheat, and small grains (Bernards et al., 2009). In a multiyear study conducted across the High Plains of the United States in a number of commercial sunflower-production fields, it was observed that approximately 66 percent of the fields existed near weedy sunflower (Burke et al., 2002). It is important that the cultivated and weedy sunflowers flowered simultaneously (52–96 percent), and evidence of hybridization ranged from 10 percent to 33 percent of the weedy sunflower (Burke et al., 2002). Evidence of genes coding for herbicide resistance in cultivated sunflower moving to weedy sunflower suggests that there is a substantial risk of the introgression of the trait into wild sunflower populations, which might result in increased management problems for growers if the wild plants are in cultivated fields (Marshall, 2001; Massinga and Al-Khatib, 2002; Massinga, 2003). These management problems may emerge in association with sunflower varieties with resistance to the herbicide imazamox that were developed using conventional breeding methods and not genetic engineering. Hybrids of imazamox-resistant sunflowers and two interbreeding relatives appear to be competitively equal to the HR domesticated sunflower, suggesting that the resistance gene will persist when gene flow occurs (Massinga et al., 2005).
GE glyphosate-resistant creeping bentgrass was field-tested in Oregon in 2003, and introgression of the transgene into weedy populations was detected at a considerable distance from the test sites (Mallory-Smith et al., 2005; Reichman et al., 2006). The inability to mitigate GE trait introgression into compatible weedy relatives of creeping bentgrass has delayed commercialization of the GE creeping bentgrass product (Charles, 2007). The transfer of this trait may be important if glyphosate is used to control weedy populations of the grass.
In the case of rice, GE glufosinate-resistant rice cultivars have been developed to improve weed management of red rice (Oryza sativa L.), a common and important weed in commercial rice production. However, these GE cultivars have never been commercially available (Gealy et al., 2007) though they were first deregulated in 1998. The commercialization of rice with resistance to the herbicide imazethapyr (produced by a chemical-induced seed mutagenesis, not genetic engineering) has resulted in the movement of this HR trait into red rice because total control of red rice is not always possible (Burgos et al., 2008). Stewardship recommendations to prevent and to control the spread of HR red rice exist and encourage crop diversity (BASF, 2009); data on the success of managing imazethapyr-resistant red rice may provide useful information for the
regulation of GE cultivars. In addition to concerns about introgression with the weed red rice, GE rice has historically been unacceptable to consumers for the reasons discussed in Chapter 1 (Gealy and Dilday, 1997; Gealy et al., 2003, 2007).
Wheat is a major grain crop in the United States, and there has been interest in commercializing genetically engineered HR cultivars. The potential for introgression of a GE trait into near-relative weed populations exists (Ellstrand et al., 1999; Morrison et al., 2002a, 2002b; Stewart et al., 2003). Jointed goatgrass (Aegilops cylindrica) is reported to be an important weed of small grains in Colorado, Kansas, New Mexico, Oklahoma, Oregon, Utah, Washington, and Wyoming (NAPPO, 2003) and is a weed in the Great Plains (Stubbendieck et al., 1994). Specifically, it causes serious problems in winter wheat in the western United States due to its similarity to wheat in appearance, seed size, growth pattern, and genetics (Schmale et al., 2008, 2009; Yenish et al., 2009). Studies have demonstrated hybridization with wheat varieties (Hanson et al., 2005; Rehman et al., 2006). It is predicted that hybridization between HR wheat cultivars and Aegilops spp. will result in the introgression of the HR trait and thus a more competitive weedy hybrid, complicating management issues in wheat production (Hanson et al., 2005; Loureiro et al., 2008). Glyphosate-resistant hard red spring wheat provided an opportunity for better weed management and resulted in a 10-percent higher grain yield than conventional wheat cultivars treated with conventional herbicides (Howatt et al., 2006). Despite the technical fit, the program to develop glyphosate-resistant cultivars was postponed in 2004 because of regulatory and marketing issues, concern for stewardship, and the inability to ensure the segregation of the GE wheat from non-GE wheat grain at the time (Dill, 2005) (see Chapter 4 for further details). An imazamox-resistant winter wheat, which was bred using conventional techniques, has been commercially available since 2002, and the identical concerns about the development of HR jointed goatgrass biotypes exist (Kniss et al., 2008).
The ecological and economic consequences of the introgression of GE traits into weedy or native relatives will vary among types of GE traits (Owen, 2008). For HR crops, the introgression of herbicide resistance from GE crops into weedy near-relatives is likely to have consequences for weed management when weeds with the resistance trait occur in fields or other ecosystems treated with the herbicide. Therefore, for future HR plants, understanding the extent to which a herbicide controls weedy relatives will provide valuable information on the consequences of gene flow for on-farm and off-farm weed management.
CONCLUSIONS
Environmental effects at the farm level have occurred as a result of the adoption of GE crops and the agricultural practices that accompany their cultivation. The introduction of GE crops has reduced pesticide use or the toxicity of pesticides used on fields where soybean, corn, and cotton are grown. Available evidence indicates that no-till practices and HR crops are complementary, and each has encouraged the other’s adoption. Conservation tillage, especially no-till, reduces soil erosion and can improve soil quality. The pesticide shifts and increase in conservation till-age with GE crops have generally benefited farmers who adopted them so far. Conservation tillage practices can also improve water quality by reducing the volume of runoff from farms into surface water, thereby reducing sedimentation and contamination from farm chemicals. Given that agriculture is the largest cause of impaired quality of surface waters, that may constitute the largest benefit of GE crops, but the infrastructure for tracking and understanding this does not exist.
The effects of Bt crops on nontarget invertebrates, including predators, are favorable or neutral, depending on the degree to which Bt crops replace insecticide treatment and on whether additional insecticide treatments are applied to the Bt crop. Evidence indicates no effect of Bt toxins on the honey bee, a widespread pollinator in agricultural systems. For HR crops, the effects on the abundance of arthropods in the fields correlate with whether weeds are controlled more effectively. Shifts in the weed communities have occurred in response to weed-management tactics used for HR crops, in particular when weeds in glyphosate-resistant crops are treated only with glyphosate. Similarly, glyphosate-resistant weeds have evolved where the glyphosate application is repeated and constitutes the only weed-management tactic used. The evolution of resistance to glyphosate in particular kinds of weeds and shifts in the weed community may increase production costs for farmers, require more tillage for weed control, and lead to at least a partial return to the use of different and often more toxic herbicides. The development and establishment of more diversified control strategies for managing weeds in HR crops is needed.
The first generation of IR crops commercialized in the United States produced a single Bt toxin for the control of insect pests. Since the commercialization of those crops, EPA has mandated the refuge strategy to delay the evolution of resistance in major insect pests that are controlled by Bt corn and cotton. After 14 years of use of Bt crops, two insect pests have evolved resistance to Bt crops in the United States: Cotton bollworm (Helicoverpa zea) evolved resistance to Cry1Ac and Cry2Ab in Bt cotton, and fall armyworm evolved resistance to Cry1F in Bt corn. The evolution in bollworm of resistance to Bt cotton did not have serious agronomic, economic, or environmental consequences. Information for assessing the
consequence of the evolution of resistance to Cry1F in Bt corn in fall army-worm is lacking. The second generation of IR crops produces two or more Bt toxins for the control of individual insect pest species. The complete replacement of one-toxin with multitoxin Bt crops should help in delaying the evolution of insect-pest resistance to IR crops.
The changes in weed and insect population densities resulting from the adoption of HR and IR crops can affect farms beyond the boundaries of the operations that are using the GE crops. That is, farming practices may have landscape as well as local level effects on pest populations. For example, large-scale planting of IR crops has decreased populations of some insect pests targeted by Bt crops not just at a farm-field level but on a regional scale. It can also affect local and possibly landscape populations of nontarget or beneficial organisms according to crop species planted and management of pests, nutrients, water, and soil (Björklund et al., 1999; Cattaneo et al., 2006; Dale and Polasky, 2007; Zhang et al., 2007; Carrière et al., 2009). Beneficial organisms with high mobility move among habitats and crop fields, so effects within a field that is planted to GE crops could influence beneficial organisms on other farms as well as noncultivated habitats in the region.
For corn and soybean, gene flow between GE varieties and wild relatives is not an issue in the United States because corn and soybean have no wild relatives here. Limited overlap occurs between cotton and wild relatives and between sugar beet and introduced, weedy relatives. Gene flow is unlikely for Bt cotton and wild relatives because of planting restrictions, but there are no planting restrictions for HR cotton. Other crops in which gene flow with wild or weedy relatives is possible include canola, alfalfa, sunflower, creeping bentgrass, wheat, and rice. Gene flow between GE and non-GE crops occurs via cross-pollination between GE and non-GE plants from different fields, co-mingling of seed before or during the production year, and germination of seeds that are left behind after the production year. Gene flow between GE and non-GE crops is almost impossible to prevent completely with current technology. The complex interactions among the multiple factors that influence gene flow between GE and non-GE crops and the resulting levels of adventitious presence of GE traits in non-GE crops deserve more attention.
REFERENCES
Abud, S., P.I.M. Souza, C.T. Moreira, A.L. Farias Neto, G.R. Vianna, S.R.M. Andrade, J. Nunes Junior, R.A. Guerzoni, P.M.F.O. Monteiro, M.S. Assuncao, E.L. Rech, and F.J.L. Aragao. 2004. Distance of flow in transgenic BROO-69515 and the non transgenic soybean in the Cerrado Region, Brasil. In Proceedings of VII World Soybean Research Conference, pp. 110–111. eds. F. Moscardi, C.B. Hoffmann-Campo, O.F. Saraiva, P.R. Galerani, F.C. Krzyzanowski, and M.C. Carrao-Panizzi. Foz do Iguassu, Brazil: Brasilian Agricultural Research Corporation.
Abud, S., P.I.M. De Souza, G.R. Vianna, E. Leonardecz, C.T. Moreira, F.G. Faleiro, J.N. Júnior, P.M.F.O. Monteiro, E.L. Rech, and F.J.L. Aragão. 2007. Gene flow from transgenic to nontransgenic soybean plants in the Cerrado region of Brazil. Genetics and Molecular Research 6(2):445–452.
Adamczyk, J.J., Jr., L.C. Adams, and D.D. Hardee. 2001. Field efficacy and seasonal expression profiles for terminal leaves of single and double Bacillus thuringiensis toxin cotton genotypes. Journal of Economic Entomology 94(6):1589–1593.
Ahl Goy, P., G. Warren, J. White, L. Privalle, P. Fearing, and D. Vlachos. 1995. Interaction of an insect tolerant maize with organisms in the ecosystem. Mitteilungen aus der Biologischen Bundesanstalt fuer Land-und Forstwirtschaft Berlin-Dahlem (309):50–53.
Ahrent, D.K., and C.E. Caviness. 1994. Natural cross-pollination of twelve soybean cultivars in Arkansas. Crop Science 34(2):376–378.
Akhurst, R.J., W. James, L.J. Bird, and C. Beard. 2003. Resistance to the Cry1Ac -endotoxin of Bacillus thuringiensis in the cotton bollworm, Helicoverpa armigera (Lepidoptera: Noctuidae). Journal of Economic Entomology 96:1290–1299.
Al-Deeb, M.A., G.E. Wilde, J.M. Blair, and T.C. Todd. 2003. Effect of Bt corn for corn rootworm control on nontarget soil microarthropods and nematodes. Environmental Entomology 32(4):859–865.
Alstad, D.N., and D.A. Andow. 1995. Managing the evolution of insect resistance to transgenic plants. Science 268(5219):1894–1896.
Andersen, N.S., H.R. Siegismund, V. Meyer, and R.B. Jørgensen. 2005. Low level of gene flow from cultivated beets (Beta vulgaris L. ssp. vulgaris) into Danish populations of sea beet (Beta vulgaris L. ssp. maritima (L.) Arcangeli). Molecular Ecology 14(5):1391–1405.
Andow, D.A., D.M. Olson, R.L. Hellmich, D.N. Alstad, and W.D. Hutchison. 2000. Frequency of resistance to Bacillus thuringiensis toxin Cry1Ab in an Iowa population of European corn borer (Lepidoptera: Crambidae). Journal of Economic Entomology 93(1):26–30.
Andow, D.A., and A.R. Ives. 2002. Monitoring and adaptive resistance management. Ecological Applications 12(5):1378–1390.
APRD (Arthropod Pesticide Resistance Database). 2009. eds. E. Whalon, D. Mota-Sanchez, R.M. Hollingworth, and L. Duynslager. Available online at http://www.pesticideresistance.com/. Accessed December 21, 2009.
Ashton, B.A., D.S. Ireland, and M.E. Westgate. 2000. Applicability of the industrial source complex (ISC) air dispersion model for use on corn pollen transport. B.Sc. thesis. Meteorology Program, Iowa State University, Ames, IA.
Bagwell, R.D., D.R. Cook, B.R. Leonard, S. Micinski, and E. Burris. 2001. Status of insecticide resistance in tobacco bollworm and bollworm in Louisiana during 2000. In Proceedings of the Beltwide Cotton Conference, pp. 785–789 (Memphis, TN, January 10–11, 2001). National Cotton Council of America.
Baker, G.H., C.R. Tann, and G.P. Fitt. 2008. Production of Helicoverpa spp. (Lepidoptera, Noctuidae) from different refuge crops to accompany transgenic cotton plantings in eastern Australia. Australian Journal of Agricultural Research 59(8):723–732.
Baker, H.G. 1991. The continuing evolution of weeds. Economic Botany 45:445–449.
BASF (Baden Aniline and Soda Factory). 2009. CLEARFIELD® rice stewardship recommendations. APN 08-10-830-0009. BASF Corporation. Research Triangle Park, NC. Available online at http://agproducts.basf.us/products/research-library/clearfield-rice-stewardship.pdf. Accessed November 11, 2009.
Baucom, R.S., and R. Mauricio. 2004. Fitness costs and benefits of novel herbicide tolerance in a noxious weed. Proceedings of the National Academy of Sciences of the United States of America 101(36):13386–13390.
Baumgarte, S., and C.C. Tebbe. 2005. Field studies on the environmental fate of the Cry1Ab Bt-toxin produced by transgenic maize (MON810) and its effect on bacterial communities in the maize rhizosphere. Molecular Ecology 14(8):2539–2551.
Beckie, H.J. 2006. Herbicide-resistant weeds: Management tactics and practices. Weed Technology 20(3):793–814.
Beckie, H.J., S.I. Warwick, H. Nair, and G. Séguin-Swartz. 2003. Gene flow in commercial fields of herbicide-resistant canola (Brassica napus). Ecological Applications 13(5):1276–1294.
Beckie, H.J., G. Séguin-Swartz, H. Nair, S.I. Warwick, and E. Johnson. 2004. Multiple herbicide-resistant canola can be controlled by alternative herbicides. Weed Science 52(1):152–157.
Beckie, H.J., K.N. Harker, L.M. Hall, S.I. Warwick, A. Légère, P.H. Sikkema, G.W. Clayton, A.G. Thomas, J.Y. Leeson, G. Séguin-Swartz, and M.J. Simard. 2006. A decade of herbicide-resistant crops in Canada. Canadian Journal of Plant Science 86(4):1243–1264.
Beekman, M., and F.L.W. Ratnieks. 2000. Long-range foraging by the honey-bee, Apis mellifera L. Functional Ecology 14(4):490–496.
Behrens, M.R., N. Mutlu, S. Chakraborty, R. Dumitru, Z.J. Wen, B.J. LaVallee, P.L. Herman, T.E. Clemente, and D.P. Weeks. 2007. Dicamba resistance: Enlarging and preserving biotechnology-based weed management strategies. Science 316(5828):1185–1188.
Bernards, M.L., R.E. Gaussoin, R.N. Klein, S.Z. Knezevic, D.J. Lyon, R.G. Wilson, P.J. Shea, and C.L. Ogg. 2009. 2009 Guide for weed management in Nebraska. EC130. University of Nebraska-Lincoln. Lincoln, NE. Available online at http://www.ianrpubs.unl.edu/sendIt/ec130.pdf. Accessed September 18, 2009.
Berwald, D., S. Matten, and D. Widawsky. 2006. Economic analysis and regulating pesticide biotechnology at the U.S. Environmental Protection Agency. In Regulating agricultural biotechnology: Economics and policy. eds. R.E. Just, J.M. Alston, and D. Zilberman, pp. 21–36. New York: Springer.
Birch, A.N.E., B.S. Griffiths, S. Caul, J. Thompson, L.H. Heckmann, P.H. Krogh, and J. Cortet. 2007. The role of laboratory, glasshouse and field scale experiments in understanding the interactions between genetically modified crops and soil ecosystems: A review of the ECOGEN project. Pedobiologia 51(3):251–260.
Björklund, J., K.E. Limburg, and T. Rydberg. 1999. Impact of production intensity on the ability of the agricultural landscape to generate ecosystem services: An example from Sweden. Ecological Economics 29(2):269–291.
Blanco-Canqui, H., and R. Lal. 2009. Crop residue removal impacts on soil productivity and environmental quality. Critical Reviews in Plant Sciences 28(3):139–163.
Boerboom, C.M. 1999. Nonchemical options for delaying weed resistance to herbicides in Midwest cropping system. Weed Technology 13:636–642.
Boerboom, C. 2005. Glyphosate-resistant common lambsquarters announced. Wisconsin Crop Manager 12(1):8–9.
Bommireddy, P.L., and B.R. Leonard. 2008. Age-specific mortality of Helicoverpa zea and Heliothis virescens (Lepidoptera: Noctuidae) larvae on flower buds of transgenic cotton expressing Vip3a and Vipcot(TM) insecticidal proteins. Journal of Entomological Science 43(4):349–361.
Borggaard, O.K., and A.L. Gimsing. 2008. Fate of glyphosate in soil and the possibility of leaching to ground and surface waters: A review. Pest Management Science 64(4):441–456.
Bourguet, D., J. Chaufaux, A. Micoud, M. Delos, B. Naibo, F. Bombarde, G. Marque, N. Eychenne, and C. Pagliari. 2002. Ostrinia nubilalis parasitism and the field abundance of non-target insects in transgenic Bacillus thuringiensis corn (Zea mays). Environmental Biosafety Research 1(1):49–60.
Bravo, A., and M. Soberón. 2008. How to cope with insect resistance to Bt toxins? Trends in Biotechnology 26(10):573–579.
Buhler, D.D. 1992. Population dynamics and control of annual weeds in corn (Zea mays) as influenced by tillage systems. Weed Science 40:241–248.
Buhler, D.D., and M.D.K. Owen. 1997. Emergence and survival of horseweed (Conyza canadensis). Weed Science 45:98–101.
Buhler, D.D., R.G. Hartzler, and F. Forcella. 1997. Implications of weed seedbank dynamics to weed management. Weed Science 45:329–336.
Burgos, N.R., J.K. Norsworthy, R.C. Scott, and K.L. Smith. 2008. Red rice (Oryza sativa) status after 5 years of imidazolinone-resistant rice technology in Arkansas. Weed Technology 22(1):200–208.
Burke, J.M., K.A. Gardner, and L.H. Rieseberg. 2002. The potential for gene flow between cultivated and wild sunflower (Helianthus annuus) in the United States. American Journal of Botany 89(9):1550–1552.
Calflora. 2009. Information on California plants for education, research and conservation. The Calflora Database. Available online at http://www.calflora.org/cgi-bin/specieslist.cgi?orderby=taxon&where-genus=Beta&ttime=1251561416&ttime=1251561417. Accessed August 29, 2009.
Carlisle, S.M., and J.T. Trevors. 1986. Effect of the herbicide glyphosate on respiration and hydrogen consumption in soil. Water, Air, and Soil Pollution 27(3–4):391–401.
Carrière, Y., and D.A. Roff. 1995. Change in genetic architecture resulting from the evolution of insecticide resistance: A theoretical and empirical analysis. Heredity 75(6):618–629.
Carrière, Y., and B.E. Tabashnik. 2001. Reversing insect adaptation to transgenic insecticidal plants. Proceedings of the Royal Society B: Biological Sciences 268(1475):1475–1480.
Carrière, Y., T.J. Dennehy, B. Pedersen, S. Haller, C. Ellers-Kirk, L. Antilla, Y.B. Liu, E. Willott, and B.E. Tabashnik. 2001. Large-scale management of insect resistance to transgenic cotton in Arizona: Can transgenic insecticidal crops be sustained? Journal of Economic Entomology 94(2):315–325.
Carrière, Y., C. Ellers-Kirk, M.S. Sisterson, L. Antilla, M. Whitlow, T.J. Dennehy, and B.E. Tabashnik. 2003. Long-term regional suppression of pink bollworm by Bacillus thuringiensis cotton. Proceedings of the National Academy of Sciences of the United States of America 100(4):1519–1523.
Carrière, Y., P. Dutilleul, C. Ellers-Kirk, B. Pedersen, S. Haller, L. Antilla, T.J. Dennehy, and B.E. Tabashnik. 2004a. Sources, sinks, and the zone of influence of refuges for managing insect resistance to Bt crops. Ecological Applications 14(6):1615–1623.
Carrière, Y., M.S. Sisterson, and B.E. Tabashnik. 2004b. Resistance management for sustainable use of Bacillus thuringiensis crops in integrated pest management. In Insect pest management: Field and protected crops. eds. A.R. Horowitz and I. Ishaaya, pp. 65–95. Berlin: Springer.
Carrière, Y., C. Ellers-Kirk, K. Kumar, S. Heuberger, M. Whitlow, L. Antilla, T.J. Dennehy, and B.E. Tabashnik. 2005. Long-term evaluation of compliance with refuge requirements for Bt cotton. Pest Management Science 61(4):327–330.
Carrière, Y., C. Ellers-Kirk, M.G. Cattaneo, C.M. Yafuso, L. Antilla, C.-Y. Huang, M. Rahman, B.J. Orr, and S.E. Marsh. 2009. Landscape effects of transgenic cotton on non-target ants and beetles. Basic and Applied Ecology 10(7):597–606.
Cattaneo, M.G., C.M. Yafuso, C. Schmidt, C.-Y. Huang, M. Rahman, C. Olson, C. Ellers-Kirk, B.J. Orr, S.E. Marsh, L. Antilla, P. Dutilleul, and Y. Carrière. 2006. Farm-scale evaluation of the impacts of transgenic cotton on biodiversity, pesticide use, and yield. Proceedings of the National Academy of Sciences of the United States of America 103(20):7571–7576.
Center for Food Safety, et al. v. Thomas J. Vilsack, et al.: Order regarding cross-motions for summary judgment. 2009. U.S. District Court for the Northern District of California. C 08-00484 JSW, Case #: Case3:08-cv-00484-JSW. Available online at http://www.earthjustice.org/library/legal_docs/9-21-09-order.pdf. Accessed December 16, 2009.
Cerdeira, A.L., and S.O. Duke. 2006. The current status and environmental impacts of glyphosate-resistant crops: A review. Journal of Environmental Quality 35(5):1633–1658.
Charles, D. 2007. Environmental regulation. U.S. courts say transgenic crops need tighter scrutiny. Science 315(5815):1069.
Clark, B.W., K.R. Prihoda, and J.R. Coats. 2006. Subacute effects of transgenic Cry1Ab Bacillus thuringiensis corn litter on the isopods Trachelipus rathkii and Armadillidium nasatum. Environmental Toxicology and Chemistry 25(10):2653–2661.
Cortet, J., M.N. Andersen, S. Caul, B. Griffiths, R. Joffre, B. Lacroix, C. Sausse, J. Thompson, and P.H. Krogh. 2006. Decomposition processes under Bt (Bacillus thuringiensis) maize: Results of a multi-site experiment. Soil Biology and Biochemistry 38(1):195–199.
Cowan, C.W., and B.D. Smith. 1993. New perspectives on a wild gourd in eastern North America. Journal of Ethnobiology 13(1):17–54.
Crickmore, N., D.R. Zeigler, J. Feitelson, E. Schnepf, J.V. Rie, D. Lereclus, J. Baum, and D.H. Dean. 2009. Bacillus thuringiensis toxin nomenclature. Brighton, UK: University of Sussex. Available online at http://www.lifesci.sussex.ac.uk/home/Neil_Crickmore/Bt/. Accessed June 26, 2009.
Crowder, D.W., and Y. Carrière. 2009. Comparing the refuge strategy for managing the evolution of insect resistance under different reproductive strategies. Journal of Theoretical Biology 261(3):423–430.
CTIC (Conservation Technology Information Center). 2009. National crop residue management survey. Available online at http://www.conservationinformation.org/?action=members_crmsurvey. Accessed October 1, 2009.
Culpepper, A.S. 2006. Glyphosate-induced weed shifts. Weed Technology 20(2):277–281.
Culpepper, A.S., and A.C. York. 2007. Glyphosate-resistant Palmer amaranth impacts southeastern agriculture. In Proceedings of the Illinois Crop Protection Technology Conference, pp. 61-63 (Champaign, IL, January 3–4, 2007). University of Illinois Urbana-Champaign. Available online at http://ipm.illinois.edu/education/proceedings/icptcp2007.pdf. Accessed June 18, 2009.
Dale, V.H., and S. Polasky. 2007. Measures of the effects of agricultural practices on ecosystem services. Ecological Economics 64(2):286–296.
Davies, T.G.E., L.M. Field, P.N.R. Usherwood, and M.S. Williamson. 2007. DDT, pyrethrins, pyrethroids and insect sodium channels. IUBMB Life 59(3):151–162.
Decker-Walters, D.S., J.E. Staub, S.-M. Chung, E. Nakata, and H.D. Quemada. 2009. Diversity in free-living populations of Cucurbita pepo (Cucurbitaceae) as assessed by random amplified polymorphic DNA. Systematic Botany 27(1):19–28.
Devare, M., L.M. Londoño-R, and J.E. Thies. 2007. Neither transgenic Bt maize (MON863) nor tefluthrin insecticide adversely affect soil microbial activity or biomass: A 3-year field analysis. Soil Biology and Biochemistry 39(8):2038–2047.
Devare, M.H., C.M. Jones, and J.E. Thies. 2004. Effect of Cry3Bb transgenic corn and tefluthrin on the soil microbial community: Biomass, activity, and diversity. Journal of Environmental Quality 33(3):837–843.
Dill, G.M. 2005. Glyphosate-resistant crops: History, status and future. Pest Management Science 61(3):219–224.
Donegan, K.K., C.J. Palm, V.J. Fieland, L.A. Porteous, L.M. Ganio, D.L. Schaller, L.Q. Bucao, and R.J. Seidler. 1995. Changes in levels, species and DNA fingerprints of soil microorganisms associated with cotton expressing the Bacillus thuringiensis var. kurstaki endotoxin. Applied Soil Ecology 2(2):111–124.
Duan, J.J., M. Marvier, J. Huesing, G. Dively, and Z.Y. Huang. 2008. A meta-analysis of effects of Bt crops on honey bees (Hymenoptera: Apidae). PLoS ONE 3(1).
Duke, S.O. 2005. Taking stock of herbicide-resistant crops ten years after introduction. Pest Management Science 61(3):211–218.
Dutton, A., H. Klein, J. Romeis, and F. Bigler. 2002. Uptake of Bt-toxin by herbivores feeding on transgenic maize and consequences for the predator Chrysoperla carnea. Ecological Entomology 27(4):441–447.
Ellstrand, N.C. 2003a. Current knowledge of gene flow in plants: Implications for transgene flow. Philosophical Transactions of the Royal Society B: Biological Sciences 358(1434):1163–1170.
———. 2003b. Dangerous liaisons? When cultivated plants mate with their wild relatives. Baltimore, MD: Johns Hopkins University Press.
Ellstrand, N.C., H.C. Prentice, and J.F. Hancock. 1999. Gene flow and introgression from domesticated plants into their wild relatives. Annual Review of Ecology and Systematics 30(1):539–563.
Estes, T.L., R. Allen, R.L. Jones, D.R. Buckler, K.H. Carr, D.I. Gustafson, C. Custin, M.J. McKee, A.G. Hornsby, and R.P. Richards. 2001. Predicted impact of transgenic crops on water quality and related ecosystems in vulnerable watersheds of the United States. In Pesticide behaviour in soils and water: Proceedings of a symposium organised by the British Crop Protection Council, pp. 357–366 (Brighton, UK, November 13–15, 2001). Farmham, Surrey, UK: British Crop Protection Council.
FAO (Food and Agriculture Organization). 2008. FAOStat: ResourceSTAT. FAO of the United Nations. Available online at http://faostat.fao.org. Accessed June 16, 2009.
Fawcett, R.S., B.R. Christensen, and D.P. Tierney. 1994. The impact of conservation tillage on pesticide runoff into surface water: A review and analysis. Journal of Soil and Water Conservation 49(2):126–135.
Feil, B., and J.E. Schmid. 2002. Dispersal of maize, wheat and rye pollen: A contribution to determining the necessary isolation distances for the cultivation of transgenic crops. Aachen, Germany: Shaker Verlag GmbH.
Fernandez-Cornejo, J., and W.D. McBride. 2002. Adoption of bioengineered crops. Agricultural Economic Report No. 810. May 1. U.S. Department of Agriculture–Economic Research Service. Washington, DC. Available online at http://www.ers.usda.gov/publications/aer810/. Accessed July 25, 2009.
Fernandez-Cornejo, J., and M.F. Caswell. 2006. The first decade of genetically engineered crops in the United States. Economic Information Bulletin No. 11. April. U.S. Department of Agriculture–Economic Research Service. Washington, DC. Available online at http://www.ers.usda.gov/publications/eib11/eib11.pdf. Accessed June 15, 2009.
Fernandez-Cornejo, J., C. Klotz-Ingram, R. Heimlich, M. Soule, W. McBride, and S. Jans. 2003. Economic and environmental impacts of herbicide tolerant and insect resistant crops in the United States. In The economic and environmental impacts of agbiotech: A global perspective. ed. N.G. Kalaitzandonakes, pp. 63–88. New York: Kluwer Academic/ Plenum Publishers.
Fernandez-Cornejo, J., R. Nehring, E.N. Sinha, A. Grube, and A. Vialou. 2009. Assessing recent trends in pesticide use in U.S. agriculture. Paper presented at the 2009 Annual Meeting of the Agricultural and Applied Economics Association (Milwaukee, WI, July 26–28, 2009). Available online at http://ageconsearch.umn.edu/handle/49271. Accessed October 1, 2009.
Ferré, J., and J. Van Rie. 2002. Biochemistry and genetics of insect resistance to Bacillus thuringiensis. Annual Review of Entomology 47(1):501–533.
Fitt, G.P. 2003. Deployment and impact of transgenic Bt cottons in Australia. In The economic and environmental impacts of agbiotech : A global perspective. ed. N.G. Kalaitzandonakes, pp. 141–164. New York: Kluwer Academic/Plenum Publishers.
FitzJohn, R., T. Armstrong, L. Newstrom-Lloyd, A. Wilton, and M. Cochrane. 2007. Hybridisation within Brassica and allied genera: Evaluation of potential for transgene escape. Euphytica 158(1):209–230.
Flores, S., D. Saxena, and G. Stotzky. 2005. Transgenic Bt plants decompose less in soil than non-Bt plants. Soil Biology and Biochemistry 37(6):1073–1082.
Folmar, L.C., H.O. Sanders, and A.M. Julin. 1979. Toxicity of the herbicide glyphosate and several of its formulations to fish and aquatic invertebrates. Archives of Environmental Contamination and Toxicology 8(3):269–278.
Foresman, C., and L. Glasgow. 2008. US grower perceptions and experiences with glyphosate-resistant weeds. Pest Management Science 64(4):388–391.
Foster, G., and S. Dabney. 1995. Agricultural tillage systems: Water erosion and sedimentation. In Farming for a better environment: A white paper. Ankeny, Iowa: Soil and Water Conservation Society.
Free, J.B. 1993. Insect pollination of crops. 2nd ed. San Diego, CA: Academic Press.
Frisvold, G., A. Boor, and J.M. Reeves. 2007. Simultaneous diffusion of herbicide tolerant cotton and conservation tillage. In Proceedings of the 2007 Beltwide Cotton Conference (New Orleans, LA, January 9–12, 2007). National Cotton Council of America.
Fuchs, M., E.M. Chirco, and D. Gonsalves. 2004a. Movement of coat protein genes from a commercial virus-resistant transgenic squash into a wild relative. Environmental Biosafety Research 3(1):5–16.
Fuchs, M., E.M. Chirco, J.R. McFerson, and D. Gonsalves. 2004b. Comparative fitness of a wild squash species and three generations of hybrids between wild virus-resistant transgenic squash. Environmental Biosafety Research 3(1):17–28.
Gahan, L.J., F. Gould, and D.G. Heckel. 2001. Identification of a gene associated with Bt resistance in Heliothis virescens. Science 293(5531):857–860.
Gassmann, A.J., Y. Carrière, and B.E. Tabashnik. 2009. Fitness costs of insect resistance to Bacillus thuringiensis. Annual Review of Entomology 54(1):147–163.
Gealy, D.R., and R.H. Dilday. 1997. Biology of red rice (Oryza sativa L.) accessions and their susceptibility to glufosinate and other herbicide. Abstract. Weed Science Society of America 37:34.
Gealy, D.R., D.H. Mitten, and J.N. Rutger. 2003. Gene flow between red rice (Oryza sativa) and herbicide-resistant rice (O. sativa): Implications for weed management. Weed Technology 17(3):627–645.
Gealy, D.R., K.J. Bradford, L. Hall, R. Hellmich, A. Raybould, J. Wolt, and D. Zilberman. 2007. Implications of gene flow in the scale-up and commercial use of biotechnology-derived crops: Economic and policy considerations. Council for Agricultural Science and Technology, December (Issue 37). Available online at http://www.cast-science.org/websiteUploads/publicationPDFs/CAST%20Issue%20Paper%2037%20galley-final-2149.pdf. Accessed October 21, 2007.
Geertson Farms Inc., et al. v. Mike Johanns, et al., and Monsanto Company: Memorandum and order Re: Permanent injunction. 2009. U.S. District Court for the Northern District of California. C 06-01075 CRB, Case #: 3:06-cv-01075-CRB. Decided May 3, 2007. Available online at http://www.aphis.usda.gov/brs/pdf/Alfalfa_Ruling_20070503.pdf. Accessed December 16, 2009.
Georghiou, G.P. 1986. The magnitude of the resistance problem. In Pesticide resistance: Strategies and tactics for management, pp. 14–43. Washington, DC: National Academy Press.
Giesy, J.P., S. Dobson, and K.R. Solomon. 2000. Ecotoxicological risk assessment for Roundup® herbicide. Reviews of Environmental Contamination and Toxicology 167:35–120.
Givnish, T.J. 2001. The rise and fall of plant species: A population biologist’s perspective. American Journal of Botany 88(10):1928–1934.
Glare, T.R., and M. O’Callaghan. 2000. Bacillus thuringiensis: Biology, ecology, and safety. New York: Wiley.
Gould, F. 1995. Comparisons between resistance management strategies for insects and weeds. Weed Technology 9(4):830–839.
Gould, F. 1998. Sustainability of transgenic insecticidal cultivars: Integrating pest genetics and ecology. Annual Review of Entomology 43:701–726.
———. 2003. Bt-resistance management—theory meets data. Nature Biotechnology 21(12):1450–1451.
Gould, F., A. Anderson, A. Jones, D. Sumerford, D.G. Heckel, J. Lopez, S. Micinski, R. Leonard, and M. Laster. 1997. Initial frequency of alleles for resistance to Bacillus thuringiensis toxins in field populations of Heliothis virescens. Proceedings of the National Academy of Sciences of the United States of America 94(8):3519–3523.
Gould, F., M.B. Cohen, J.S. Bentur, G.G. Kennedy, and J. Van Duyn. 2006. Impact of small fitness costs on pest adaptation to crop varieties with multiple toxins: A heuristic model. Journal of Economic Entomology 99(6):2091–2099.
Green, J.M. 2007. Review of glyphosate and ALS-inhibiting herbicide crop resistance and resistant weed management. Weed Technology 21(2):547–558.
Green, J.M. 2009. Evolution of glyphosate-resistant crop technology. Weed Science 57(1):108–117.
Green, J.M., C.B. Hazel, D.R. Forney, and L.M. Pugh. 2008. New multiple-herbicide crop resistance and formulation technology to augment the utility of glyphosate. Pest Management Science 64(4):332–339.
Greene, J.K., S.G. Turnipseed, M.J. Sullivan, and O.L. May. 2001. Treatment thresholds for stink bugs (Hemiptera: Pentatomidae) in cotton. Journal of Economic Entomology 94(2):403–409.
Greene, J.K., S. Bundy, P.M. Roberts, and B.R. Leonard. 2006. Identification and management of common boll-feeding bugs in cotton. August. EB-158. Clemson University. Blackville, SC. Available online at http://www.clemson.edu/psapublishing/PAGES/ENTOM/EB158.pdf. Accessed February 26, 2009.
Gressel, J. 1996. Fewer constraints than proclaimed to the evolution of glyphosate-resistant weeds. Resistant Pest Management 8(2):2–5.
Gressel, J., and L.A. Segel. 1990. Modeling the effectiveness of herbicide rotations and mixtures as strategies to delay or preclude resistance. Weed Technology 4:186–198.
Griffiths, B.S., S. Caul, J. Thompson, A.N.E. Birch, C. Scrimgeour, M.N. Andersen, J. Cortet, A. Messéan, C. Sausse, B. Lacroix, and P.H. Krogh. 2005. A comparison of soil microbial community structure, protozoa and nematodes in field plots of conventional and genetically modified maize expressing the Bacillus thuringiensis Cry1Ab toxin. Plant and Soil 275(1–2):135–146.
Griffiths, B.S., S. Caul, J. Thompson, A.N.E. Birch, C. Scrimgeour, J. Cortet, A. Foggo, C.A. Hackett, and P.H. Krogh. 2006. Soil microbial and faunal community responses to Bt maize and insecticide in two soils. Journal of Environmental Quality 35(3):734–741.
Gustafson, D.I. 2008. Sustainable use of glyphosate in North American cropping systems. Pest Management Science 64(4):409–416.
Hanson, B.D., C.A. Mallory-Smith, W.J. Price, B. Shafii, D.C. Thill, and R.S. Zemetra. 2005. Interspecific hybridization: Potential for movement of herbicide resistance from wheat to jointed goatgrass (Aegilops cylindrica). Weed Technology 19(3):674–682.
Hanson, J.D., J. Hendrickson, and D. Archer. 2008. Challenges for maintaining sustainable agricultural systems in the United States. Renewable Agriculture and Food Systems 23(4):325–334.
Hawes, C., A.J. Haughton, J.L. Osborne, D.B. Roy, S.J. Clark, J.N. Perry, P. Rothery, D.A. Bohan, D.R. Brooks, G.T. Champion, A.M. Dewar, M.S. Heard, I.P. Woiwod, R.E. Daniels, M.W. Young, A.M. Parish, R.J. Scott, L.G. Firbank, and G.R. Squire. 2003. Responses of plants and invertebrate trophic groups to contrasting herbicide regimes in the farm scale evaluations of genetically modified herbicide-tolerant crops. Philosophical Transactions of the Royal Society B: Biological Sciences 358(1439):1899–1913.
Hawes, C., A.J. Haughton, D.A. Bohan, and G.R. Squire. 2009. Functional approaches for assessing plant and invertebrate abundance patterns in arable systems. Basic and Applied Ecology 10(1):34–42.
Head, G., W. Moar, M. Eubanks, B. Freeman, J. Ruberson, A. Hagerty, and S. Turnipseed. 2005. A multiyear, large-scale comparison of arthropod populations on commercially managed Bt and non-Bt cotton fields. Environmental Entomology 34(5):1257–1266.
Heap, I. 2010. The international survey of herbicide resistant weeds. Available online at http://www.weedscience.org/. Accessed March 31, 2010.
Hernández-Martínez, P., J. Ferré, and B. Escriche. 2009. Broad-spectrum cross-resistance in Spodoptera exigua from selection with a marginally toxic Cry protein. Pest Management Science 65(6):645–650.
Heuberger, S., and Y. Carrière. 2009. Pollen-mediated transgene flow in agricultural seed production. Paper presented at the 94th Ecological Society of America annual meeting (Albuquerque, NM, August 2–7, 2009).
Heuberger, S., C. Ellers-Kirk, C. Yafuso, A.J. Gassmann, B.E. Tabashnik, T.J. Dennehy, and Y. Carrière. 2009. Effects of refuge contamination by transgenes on Bt resistance in pink bollworm (Lepidoptera: Gelechiidae). Journal of Economic Entomology 101(2):504–514.
Hilgenfeld, K.L., A.R. Martin, D.A. Mortensen, and S.C. Mason. 2004. Weed management in a glyphosate resistant soybean system: Weed species shifts. Weed Technology 18(2):284–291.
Holland, J.M. 2004. The environmental consequences of adopting conservation tillage in Europe: Reviewing the evidence. Agriculture, Ecosystems and Environment 103(1):1–25.
Hopkins, D.W., and E.G. Gregorich. 2003. Detection and decay of the Bt endotoxin in soil from a field trial with genetically modified maize. European Journal of Soil Science 54(4):793–800.
Howatt, K.A. 2008. Personal communication to M.D.K. Owen.
Howatt, K.A., G.J. Endres, P.E. Hendrickson, E.Z. Aberle, J.R. Lukach, B.M. Jenks, N.R. Riveland, S.A. Valenti, and C.M. Rystedt. 2006. Evaluation of glyphosate-resistant hard red spring wheat (Triticum aestivum). Weed Technology 20:706–716.
Hurley, T.M., and P.D. Mitchell. 2008. Insect resistance management: Adoption and compliance. In Insect resistance management: Biology, economics, and prediction. ed. D.W. Onstad, pp. 227–253. San Diego, CA: Elselvier.
Hutchison, W.D., E.C. Burkness, R.D. Moon, T. Leslie, S.J. Fleischer, and M. Abrahamson. 2007. Evidence for regional suppression of European corn borer populations in trans-genic Bt maize in the Midwestern USA: Analysis of long-term time series data from three states, Vol. 2. In Proceedings of the XVI International Plant Protection Congress, pp. 512-513 (Glasgow, UK, October 15–18, 2007). British Crop Production Council.
Icoz, I., and G. Stotzky. 2008. Fate and effects of insect-resistant Bt crops in soil ecosystems. Soil Biology and Biochemistry 40(3):559–586.
Icoz, I., D. Saxena, D.A. Andow, C. Zwahlen, and G. Stotzky. 2008. Microbial populations and enzyme activities in soil in situ under transgenic corn expressing cry proteins from Bacillus thuringiensis. Journal of Environmental Quality 37(2):647–662.
Jackson, R.E., and H.N. Pitre. 2004. Influence of Roundup Ready® soybean production systems and glyphosate application on pest and beneficial insects in wide-row soybean. Journal of Agricultural and Urban Entomology 21(2):61–70.
Jackson, R.E., J.R. Bradley Jr., and J.W. Van Duyn. 2003. Field performance of transgenic cottons expressing one or two Bacillus thuringiensis endotoxins against bollworm, Helicoverpa zea (Boddie). Journal of Cotton Science 7(3):57–64.
Jaffe, G. 2009. Complacency on the farm: Significant noncompliance with EPA’s refuge requirements threatens the future effectiveness of genetically engineered pest-protected corn. November. Center for Science in the Public Interest. Washington, DC. Available online at http://cspinet.org/new/pdf/complacencyonthefarm.pdf. Accessed August 5, 2009.
Jasieniuk, M., A.L. Brûlé-Babel, and I.N. Morrison. 1996. The evolution and genetics of herbicide resistance in weeds. Weed Science 44(1):176–193
Johnson, W.G., and K.D. Gibson. 2006. Glyphosate-resistant weeds and resistance management strategies: An Indiana grower perspective. Weed Technology 20(3):768–772.
Johnson, W.G., M.D.K. Owen, G.R. Kruger, B.G. Young, D.R. Shaw, R.G. Wilson, J.W. Wilcut, D.L. Jordan, and S.C. Weller. 2009. U.S. farmer awareness of glyphosate-resistant weeds and resistance management strategies. Weed Technology 23(2):308–312.
Jung, H.G., and C.C. Sheaffer. 2004. Influence of Bt transgenes on cell wall lignification and digestibility of maize stover for silage. Crop Science 44(5):1781–1789.
Kalaitzandonakes, N., and P. Suntornpithug. 2003. Adoption of cotton biotechnology in the United States: Implications for impact assessment. In The economic and environmental impacts of agbiotech: A global perspective. ed. N.G. Kalaitzandonakes, pp. 103–118. New York: Kluwer Academic/Plenum Publishers.
Keeler, K.H., C.E. Turner, and M.R. Bolick. 1996. Movement of crop transgenes into wild plants. In Herbicide-resistant crops: Agricultural, environmental, economic, regulatory, and technical aspects. ed. S.O. Duke, pp. 303–330. Boca Raton: Lewis Publishers.
Kennedy, G.G., and N.P. Storer. 2000. Life systems of polyphagous arthropod pests in temporally unstable cropping systems. Annual Review of Entomology 45:467–493.
Kennedy, G.G., F. Gould, O.M.B. Deponti, and R.E. Stinner. 1987. Ecological, agricultural, genetic, and commercial considerations in the deployment of insect-resistant germplasm. Environmental Entomology 16(2):327–338.
Kirkpatrick, K.J., and H.D. Wilson. 1988. Interspecific gene flow in Cucurbita: C. texana vs. C. pepo. American Journal of Botany 75(4):519–527.
Kladivko, E.J. 2001. Tillage systems and soil ecology. Soil and Tillage Research 61(1-2):61–76.
Knispel, A.L., S.M. McLachlan, R.C. Van Acker, and L.F. Friesen. 2008. Gene flow and multiple herbicide resistance in escaped canola populations. Weed Science 56(1):72–80.
Kniss, A.R., S.D. Miller, and R.G. Wilson. 2004. Factors influencing common lambsquarters control with glyphosate. In Proceedings of the 59th Annual Meeting of the North Central Weed Science Society, p. 85 (Columbus, OH, December 13–16, 2004). ed. R.G. Hartzler. North Central Weed Science Society. Available online at http://www.ncwss.org/proceed/2004/proc04/abstracts/085.pdf. Accessed January 31, 2009.
———. 2005. Common lambsquarters control with glyphosate: What’s the problem? In Proceedings of the 60th Annual Meeting of the North Central Weed Science Society, p. 200 (Kansas City, MO, December 12–15, 2005). ed. R.G. Hartzler. North Central Weed Science Society. Available online at http://www.ncwss.org/proceed/2005/proc05/abstracts/200.pdf. Accessed July 24, 2009.
Kniss, A.R., D.J. Lyon, and S.D. Miller. 2008. Jointed goatgrass management with imazamoxresistant cultivars in a winter wheat-fallow rotation. Crop Science 48(6):2414–2420.
Kolpin, D.W., E.M. Thurman, E.A. Lee, M.T. Meyer, E.T. Furlong, and S.T. Glassmeyer. 2006. Urban contributions of glyphosate and its degradate AMPA to streams in the United States. Science of the Total Environment 354(2-3):191–197.
Kravchenko, A.N., X. Hao, and G.P. Robertson. 2009. Seven years of continuously planted Bt corn did not affect mineralizable and total soil C and total N in surface soil. Plant and Soil 318(1–2):269–274.
Kremer, R.J., and N.E. Means. 2009. Glyphosate and glyphosate-resistant crop interactions with rhizosphere microorganisms. European Journal of Agronomy 31(3):153–161.
Krogh, P.H., B. Griffiths, D. Demašar, M. Bohanec, M. Debeljak, M.N. Andersen, C. Sausse, A.N.E. Birch, S. Caul, M. Holmstrup, L.H. Heckmann, and J. Cortet. 2007. Responses by earthworms to reduced tillage in herbicide tolerant maize and Bt maize cropping systems. Pedobiologia 51(3):219–227.
Kruger, M., J.B.J. Van Rensburg, and J. Van den Berg. 2009. Perspective on the development of stem borer resistance to Bt maize and refuge compliance at the Vaalharts irrigation scheme in South Africa. Crop Protection 28(8):684–689.
Krupke, C., P. Marquardt, W. Johnson, S. Weller, and S.P. Conley. 2009. Volunteer corn presents new challenges for insect resistance management. Agronomy Journal 101(4):797–799.
Lachnicht, S.L., P.F. Hendrix, R.L. Potter, D.C. Coleman, and D.A. Crossley Jr. 2004. Winter decomposition of transgenic cotton residue in conventional-till and no-till systems. Applied Soil Ecology 27(2):135–142.
Landis, D.A., M.M. Gardiner, W. Van Der Werf, and S.M. Swinton. 2008. Increasing corn for biofuel production reduces biocontrol services in agricultural landscapes. Proceedings of the National Academy of Sciences of the United States of America 105(51):20552–20557.
Lang, A., R. Beck, J. Bauchhenß, G. Pommer, and M. Arndt. 2006. Monitoring of the environmental effects of the Bt gene. 10/2006. Bavarian State Research Center for Agriculture. August ed. Freising, Germany. Available online at http://www.lfl-neu.bayern.de/publikationen/daten/schriftenreihe_url_1_43.pdf. Accessed April 12, 2009.
Laughlin, K.D., A.G. Power, A.A. Snow, and L.J. Spencer. 2009. Risk assessment of genetically engineered crops: Fitness effects of virus-resistance transgenes in wild Cucurbita pepo. Ecological Applications 19(5):1091–1101.
Leer, S. 2006. Glyphosate-resistant giant ragweed confirmed in Indiana, Ohio. Purdue University Newsletter, December 21. Available online at http://www.agriculture.purdue.edu/agcomm/agnews/public/story.asp?newsid=1594. Accessed April 12, 2009.
Légère, A. 2005. Risks and consequences of gene flow from herbicide-resistant crops: Canola (Brassica napus L) as a case study. Pest Management Science 61(3):292–300.
Legleiter, T.R., and K.W. Bradley. 2008. Glyphosate and multiple herbicide resistance in common waterhemp (Amaranthus rudis) populations from Missouri. Weed Science 56(4):582–587.
Lehman, R.M., S.L. Osborne, and K.A. Rosentrater. 2008. No differences in decomposition rates observed between Bacillus thuringiensis and non-Bacillus thuringiensis corn residue incubated in the field. Agronomy Journal 100(1):163–168.
Liebig, M.A., D.L. Tanaka, and B.J. Wienhold. 2004. Tillage and cropping effects on soil quality indicators in the northern Great Plains. Soil and Tillage Research 78(2):131–141.
Liphadzi, K.B., K. Al-Khatib, C.N. Bensch, P.W. Stahlman, J.A. Dille, T. Todd, C.W. Rice, M.J. Horak, and G. Head. 2005. Soil microbial and nematode communities as affected by glyphosate and tillage practices in a glyphosate-resistant cropping system. Weed Science 53(4):536–545.
Liu, Y.B., B.E. Tabashnik, and M. Pusztai-Carey. 1996. Field-evolved resistance to Bacillus thuringiensis toxin Cry1C in diamondback moth (Lepidoptera: Plutellidae). Journal of Economic Entomology 89(4):798–804.
Llewellyn, D., C. Tyson, G. Constable, B. Duggan, S. Beale, and P. Steel. 2007. Containment of regulated genetically modified cotton in the field. Agriculture, Ecosystems and Environment 121(4):419–429.
Locke, M.A., and C.T. Bryson. 1997. Herbicide-soil interactions in reduced tillage and plant residue management systems. Weed Science 45(2):307–320.
Locke, M.A., R.M. Zablotowicz, and K.N. Reddy. 2008. Integrating soil conservation practices and glyphosate-resistant crops: Impacts on soil. Pest Management Science 64(4):457–469.
Loureiro, I., C. Escorial, J.-M. Garcia Baudin, and M.-C. Chueca. 2008. Hybridisation between wheat and Aegilops geniculata and hybrid fertility for potential herbicide resistance transfer. Weed Research 48:561–570.
Luna V., S., J. Figueroa M., B. Baltazar M., R. Gomez L., R. Townsend, and J.B. Schoper. 2001. Maize pollen longevity and distance isolation requirements for effective pollen control. Crop Science 41(5):1551–1557.
Mahon, R.J., and K.M. Olsen. 2009. Limited survival of a Cry2Ab-resistant strain of Helicoverpa armigera (Lepidoptera: Noctuidae) on Bollgard II. Journal of Economic Entomology 102(2):708–716.
Mahon, R.J., K.M. Olsen, S. Downes, and S. Addison. 2007. Frequency of alleles conferring resistance to the Bt toxins Cry1Ac and Cry2Ab in Australian populations of Helicoverpa armigera (Lepidoptera: Noctuidae). Journal of Economic Entomology 100(6):1844–1853.
Malik, J., G. Barry, and G. Kishore. 1989. The herbicide glyphosate. Biofactors 2(1):17–25.
Mallory-Smith, C., M. Butler, and C. Campbell. 2005. Gene movement from glyphosate-resistant creeping bentgrass (Agrostis stolonifera) fields, Abstracts, Vol. 45. In Proceedings of the Weed Science Society of America, pp. 49–50 (Honolulu, HI).
Manachini, B. 2003. Effect of transgenic corn on Lydella thompsoni Hertig (Diptera: Tachinidae) parasitoid of Ostrinia nubilalis Hb. (Lepidoptera: Crambidae). Bollettino di Zoologia Agraria e di Bachicoltura 35(111–125).
Marshall, M.W., K. Al-Khatib, and T. Loughin. 2001. Gene flow, growth, and competitiveness of imazethapyr-resistant common sunflower. Weed Science 49:14–21.
Marvier, M., C. McCreedy, J. Regetz, and P. Kareiva. 2007. A meta-analysis of effects of Bt cotton and maize on nontarget invertebrates. Science 316(5830):1475–1477.
Marvier, M., Y. Carrière, N. Ellstrand, P. Gepts, P. Kareiva, E. Rosi-Marshall, B.E. Tabashnik, and L.L. Wolfenbarger. 2008. Harvesting data from genetically engineered crops. Science 320(5875):452–453.
Massinga, R.A., K. Al-Khatib, P. St. Amand, and J.F. Miller. 2005. Relative fitness of imazamox-resistant common sunflower and prairie sunflower. Weed Science 53(2):166–174.
Massinga, R.A., and K. Al-Khatib. 2002. Gene flow of imidazolinone resistance from cultivated sunflower to common sunflower (Helianthus annuus) and prairie sunflower (H. petiolaris). In Proceedings of the Weed Science Society of America,Vol. 42. p. 21 (Reno, NV). ed. J. Wilcut. Weed Science Society of America.
Massinga, R.A., K. Al-Khatib, P. St. Amand, and J. F. Miller. 2003. Gene flow from imidazolinone-resistant domesticated sunflower to wild relatives. Weed Science 51:854–862.
Matten, S.R., G.P. Head, and H.D. Quemada. 2008. How governmental regulation can help or hinder the integration of Bt crops into IPM programs. In Integration of insect-resistant genetically modified crops within IPM programs, Progress in biological control. Vol. 5. eds. J. Romeis, A.M. Shelton, and G.G. Kennedy, pp. 27–39. New York: Springer.
Matus-Cádiz, M.A., P. Hucl, M.J. Horak, and L.K. Blomquist. 2004. Gene flow in wheat at the field scale. Crop Science 44(3):718–727.
Maxwell, B., and M. Jasieniuk. 2000. The evolution of herbicide resistance evolution models. In Proceedings of the 3rd International Weed Science Congress, p. 172 (Foz do Iguassu, Brazil, June 6–11, 2000). International Weed Science Society. Available online at http://www.iwss.info/docs/IWSC_2000.pdf. Accessed August 9, 2009.
McGregor, S.E. 1976. Insect pollination of cultivated crop plants. U.S. Department of Agriculture–Agricultural Research Service. Tucson, AZ. Available online at http://gears.tucson.ars.ag.gov/book/. Accessed July 1, 2009.
McKenzie, J.A. 1996. Ecological and evolutionary aspects of insecticide resistance. Austin, TX: R.G. Landes and Academic Press.
Men, X., F. Ge, C.A. Edwards, and E.N. Yardim. 2005. The influence of pesticide applications on Helicoverpa armigera Hübner and sucking pests in transgenic Bt cotton and non-transgenic cotton in China. Crop Protection 24(4):319–324.
Mendelsohn, M., J. Kough, Z. Vaituzis, and K. Matthews. 2003. Are Bt crops safe? Nature Biotechnology 21(9):1003–1009.
Mensah, E.C. 2007. Economics of technology adoption: A simple approach. Saarbrücken, Germany: VDM Verlag.
Micinski, S., D.C. Blouin, W.F. Waltman, and C. Cookson. 2008. Abundance of Helicoverpa zea and Heliothis virescens in pheromone traps during the past twenty years in North-western Louisiana. Southwestern Entomologist 33(2):139–149.
Mickelson, S.K., P. Boyd, J.L. Baker, and S.I. Ahmed. 2001. Tillage and herbicide incorporation effects on residue cover, runoff, erosion, and herbicide loss. Soil and Tillage Research 60(1–2):55–66.
Mitchell, P.D., and D.W. Onstad. 2005. Effect of extended diapause on evolution of resistance to transgenic Bacillus thuringiensis corn by northern corn rootworm (Coleoptera: Chrysomelidae). Journal of Economic Entomology 98(6):2220–2234.
Moar, W.J., M. Pusztai-Carey, H.V. Faassen, D. Bosch, R. Frutos, C. Rang, K. Luo, and M.J. Adang. 1995. Development of Bacillus thuringiensis Cry1C resistance by Spodoptera exigua (Hubner) (Lepidoptera: Noctuidae). Applied and Environmental Microbiology 61(6):2086–2092.
Moar, W., R. Roush, A. Shelton, J. Ferré, S. MacIntosh, B.R. Leonard, and C. Abel. 2008. Field-evolved resistance to Bt toxins. Nature Biotechnology 26(10):1072–1074.
Morin, S., R.W. Biggs, M.S. Sisterson, L. Shriver, C. Ellers-Kirk, D. Higginson, D. Holley, L.J. Gahan, D.G. Heckel, Y. Carrière, T.J. Dennehy, J.K. Brown, and B.E. Tabashnik. 2003. Three cadherin alleles associated with resistance to Bacillus thuringiensis in pink bollworm. Proceedings of the National Academy of Sciences of the United States of America 100(9):5004–5009.
Morrison, L.A., C.C. Lisele, and C.A. Mallory-Smith. 2002a. Infestations of jointed goatgrass (Aegilops cylindrica) and its hybrids with wheat in Oregon wheat fields. Weed Science 50:737–747.
Morrison, L.A., O. Riera-Lizarazu, L. Cremieux, and C.A. Mallory-Smith. 2002b. Jointed goatgrass (Aegilops cylindrica Host) X wheat (Triticum aestivum L.) hybrids: Hybridization dynamics in Oregon wheat fields. Crop Science 42:1863–1872.
Mueller, T.C., P.D. Mitchell, B.G. Young, and A.S. Culpepper. 2005. Proactive versus reactive management of glyphosate-resistant or -tolerant weeds. Weed Technology 19(4):924–933.
Murphy, C.E., and D. Lemerle. 2006. Continuous cropping systems and weed selection. Euphytica 148(1–2):61–73.
NAPPO (North American Plant Protection Organization). 2003. Aegilops cylindrica Host. PRA/ Grains panel pest facts sheet. North American Plant Protection Organization. Ottawa, Ontario. Available online at http://www.nappo.org/PRA-sheets/Aegilopscylindrica.pdf. Accessed November 6, 2009.
Naranjo, S.E. 2005. Long-term assessment of the effects of transgenic Bt cotton on the function of the natural enemy community. Environmental Entomology 34(5):1211–1223.
———. 2009. Impacts of Bt crops on non-target invertebrates and insecticide use patterns. Perspectives in Agriculture, Veterinary Science, Nutrition and Natural Resources 4(11):1–11.
Neve, P. 2008. Simulation modelling to understand the evolution and management of glyphosate resistance in weeds. Pest Management Science 64(4):392–401.
Neve, P., A.J. Diggle, F.P. Smith, and S.B. Powles. 2003. Simulating evolution of glyphosate resistance in Lolium rigidum I: Population biology of a rare resistance trait. Weed Research 43(6):404–417.
NRC (National Research Council). 2002. Environmental effects of transgenic plants: The scope and adequacy of regulation. Washington, DC: National Academy Press.
Oliver, L.R., S.A. Harrison, and M. McClelland. 1983. Germination of Texas gourd (Cucurbita texana) and its control in soybeans (Glycine max). Weed Science 31(5):700–706.
Olsen, K.M., J.C. Daly, H.E. Holt, and E.J. Finnegan. 2005. Season-long variation in expression of Cry1Ac gene and efficacy of Bacillus thuringiensis toxin in transgenic cotton against Helicoverpa armigera (Lepidoptera: Noctuidae). Journal of Economic Entomology 98(3):1007–1017.
Onstad, D.W. 2008. Insect resistance management: Biology, economics, and prediction. San Diego, CA: Elselvier.
Orr, D.B., and D.A. Landis. 1997. Oviposition of European corn borer (Lepidoptera: Pyralidae) and impact of natural enemy populations in transgenic versus isogenic corn. Journal of Economic Entomology 90(4):905–909.
Owen, M.D.K. 2001. Importance of weed population shifts and herbicide resistance in the Midwest USA Corn Belt. In Pesticide behaviour in soils and water: Proceedings of a symposium organised by the British Crop Protection Council, pp. 407–412 (Brighton, UK, November 13–15, 2001). Farmham, Surrey, UK: British Crop Protection Council.
———. 2008. Weed species shifts in glyphosate-resistant crops. Pest Management Science 64(4):377–387.
Owen, M.D.K., and I.A. Zelaya. 2005. Herbicide-resistant crops and weed resistance to herbicides. Pest Management Science 61(3):301–311.
Ozinga, W.A., R.M. Bekker, J.H.J. Schaminée, and J.M. Van Groenendael. 2004. Dispersal potential in plant communities depends on environmental conditions. Journal of Ecology 92(5):767–777.
Palmer, R.G., J. Gai, H. Sun, and J.W. Burton. 2001. Production and evaluation of hybrid soybean. In Plant breeding reviews, Vol. 20. ed. J. Janick, pp. 263-308. New York: John Wiley & Sons, Inc.
Palumbi, S.R. 2001. Humans as the world’s greatest evolutionary force. Science 293(5536):1786–1790.
Patzoldt, W.L., P.J. Tranel, and A.G. Hager. 2005. A waterhemp (Amaranthus tuberculatus) biotype with multiple resistance across three herbicide sites of action. Weed Science 53(1):30–36.
Pereira, E.J.G., N.P. Storer, and B.D. Siegfried. 2008. Inheritance of Cry1F resistance in laboratory-selected European corn borer and its survival on transgenic corn expressing the Cry1F toxin. Bulletin of Entomological Research 98(6):621–629.
Peterson, R.K.D., and A.G. Hulting. 2004. A comparative ecological risk assessment for herbicides used on spring wheat: The effect of glyphosate when used within a glyphosate-tolerant wheat system. Weed Science 52(5):834–844.
Pietrantonio, P.V., T.A. Junek, R. Parker, D. Mott, K. Siders, N. Troxclair, J. Vargas-Camplis, J.K. Westbrook, and V.A. Vassiliou. 2007. Detection and evolution of resistance to the pyrethroid cypermethrin in Helicoverpa zea (Lepidoptera: Noctuidae) populations in Texas. Environmental Entomology 36(5):1174–1188.
Poerschmann, J., A. Gathmann, J. Augustin, U. Langer, and T. Górecki. 2005. Molecular composition of leaves and stems of genetically modified Bt and near-isogenic non-Bt maize—characterization of lignin patterns. Journal of Environmental Quality 34(5):1508–1518.
Pons, X., and P. Starý. 2003. Spring aphid-parasitoid (Hom., Aphididae, Hym., Braconidae) associations and interactions in a Mediterranean arable crop ecosystem, including Bt maize. Anzeiger fur Schadlingskunde 76(5):133–138.
Powles, S.B. 2008. Evolved glyphosate-resistant weeds around the world: Lessons to be learnt. Pest Management Science 64(4):360–365.
Powles, S.B., and C. Preston. 2006. Evolved glyphosate resistance in plants: Biochemical and genetic basis of resistance. Weed Technology 20(2):282–289.
Powles, S.B., D.F. Lorraine-Colwill, J.J. Dellow, and C. Preston. 1998. Evolved resistance to glyphosate in rigid ryegrass (Lolium rigidum) in Australia. Weed Science 46(5):604–607.
Puricelli, E., and D. Tuesca. 2005. Weed density and diversity under glyphosate-resistant crop sequences. Crop Protection 24(6):533–542.
Quemada, H., L. Strehlow, D. Decker-Walters, S., and J. Staub, E. 2008. Population size and incidence of virus infection in free-living populations of Cucurbita pepo. Environmental Biosafety Research 7(4):185–196.
Rehman, M., J.L. Hansen, J. Brown, W. Price, R.S. Zemetra, and C.A. Mallory-Smith. 2006. Effect of wheat genotype on the phenotype of wheat x jointed goatgrass (Aegilops cylindrica) hybrids. Weed Science 54(4):690–694.
Reichman, J.R., L.S. Watrud, E.H. Lee, C.A. Burdick, M.A. Bollman, M.J. Storm, G.A. King, and C. Mallory-Smith. 2006. Establishment of transgenic herbicide-resistant creeping bentgrass (Agrostis stolonifera L.) in nonagronomic habitats. Molecular Ecology 15(13):4243–4255.
Relyea, R.A., and D.K. Jones. 2009. The toxicity of Roundup Original Max® to 13 species of larval amphibians. Environmental Toxicology and Chemistry 28(9):2004–2008.
Riggin-Bucci, T.M., and F. Gould. 1997. Impact of intraplot mixtures of toxic and nontoxic plants on population dynamics of diamondback moth (Lepidoptera: Plutellidae) and its natural enemies. Journal of Economic Entomology 90(2):241–251.
Roberts, R.K., B.C. English, Q. Gao, and J.A. Larson. 2006. Simultaneous adoption of herbicide-resistance and conservation-tillage cotton technologies. Journal of Agricultural and Applied Economics 38(3):629–643.
Romeis, J., M. Meissle, and F. Bigler. 2006. Transgenic crops expressing Bacillus thuringiensis toxins and biological control. Nature Biotechnology 24(1):63–71.
Rosi-Marshall, E.J., J.L. Tank, T.V. Royer, M.R. Whiles, M. Evans-White, C. Chambers, N.A. Griffiths, J. Pokelsek, and M.L. Stephen. 2007. Toxins in transgenic crop byproducts may affect headwater stream ecosystems. Proceedings of the National Academy of Sciences of the United States of America 104(41):16204–16208.
Roush, R. 1997. Managing resistance to transgenic crops. In Advances in insect control: The role of transgenic plants. eds. N. Carozzi and M. Koziel, pp. 271–294. London: Taylor & Francis Ltd.
Roush, R.T. 1998. Two-toxin strategies for management of insecticidal transgenic crops: Can pyramiding succeed where pesticide mixtures have not? Philosophical Transactions of the Royal Society B: Biological Sciences 353(1376):1777–1786.
Roux, F., M. Paris, and X. Reboud. 2008. Delaying weed adaptation to herbicide by environmental heterogeneity: A simulation approach. Pest Management Science 64(1):16–29.
Sammons, R.D., D.C. Heering, N. Dinicola, H. Glick, and G.A. Elmore. 2007. Sustainability and stewardship of glyphosate and glyphosate-resistant crops. Weed Technology 21(2):347–354.
Sasu, M.A., M.J. Ferrari, D. Du, J.A. Winsor, and A.G. Stephenson. 2009. Indirect costs of a nontarget pathogen mitigate the direct benefits of a virus-resistant transgene in wild Cucurbita. Proceedings of the National Academy of Sciences of the United States of America 106(45):19067–19071.
Saxena, D., and G. Stotzky. 2001a. Bacillus thuringiensis (Bt) toxin released from root exudates and biomass of Bt corn has no apparent effect on earthworms, nematodes, protozoa, bacteria, and fungi in soil. Soil Biology and Biochemistry 33(9):1225–1230.
———. 2001b. Bt corn has a higher lignin content than non-Bt corn. American Journal of Botany 88(9):1704–1706.
Schmale, D., R. Anderson, D. Lyon, and B. Klein. 2008. Jointed goatgrass: Best management practices, central Great Plains. EB2033E. Washington State University. Pullman, WA. Available online at http://cru.cahe.wsu.edu/CEPublications/eb2033e/eb2033e.pdf. Accessed August 5, 2009.
Schmale, D., T. Peeper, and P. Stahlman. 2009. Jointed goatgrass: Best management practices, southern Great Plains. EM011. Washington State University. Pullman, WA. Available online at http://jointedgoatgrass.wsu.edu/jointedgoatgrass/bulletins/EM011_Final_Version.pdf. Accessed September 14, 2009.
Schnepf, E., N. Crickmore, J. Van Rie, D. Lereclus, J. Baum, J. Feitelson, D.R. Zeigler, and D.H. Dean. 1998. Bacillus thuringiensis and its pesticidal crystal proteins. Microbiology and Molecular Biology Reviews 62(3):775–806.
Schuler, T.H., R.P.J. Potting, I. Denholm, S.J. Clark, A.J. Clark, C.N. Stewart, and G.M. Poppy. 2003. Tritrophic choice experiments with Bt plants, the diamondback moth (Plutella xylostella) and the parasitoid Cotesia plutellae. Transgenic Research 12(3):351–361.
Schuler, T.H., I. Denholm, S.J. Clark, C.N. Stewart, and G.M. Poppy. 2004. Effects of Bt plants on the development and survival of the parasitoid Cotesia plutellae (Hymenoptera: Braconidae) in susceptible and Bt-resistant larvae of the diamondback moth, Plutella xylostella (Lepidoptera: Plutellidae). Journal of Insect Physiology 50(5):435–443.
Schuster, C.L., D.E. Shoup, and K. Al-Khatib. 2007. Response of common lambsquarters (Chenopodium album) to glyphosate as affected by growth stage. Weed Science 55(2):147–151.
Scott, B.A., and M.J. VanGessel. 2007. Delaware soybean grower survey on glyphosate-resistant horseweed (Conyza canadensis). Weed Technology 21(1):270–274.
Scursoni, J.A., F. Forcella, and J. Gunsolus. 2007. Weed escapes and delayed weed emergence in glyphosate-resistant soybean. Crop Protection 26(3):212–218.
Shaner, D.L. 2000. The impact of glyphosate-tolerant crops on the use of other herbicides and on resistance management. Pest Management Science 56(4):320–326.
Shelton, A.M., S.L. Hatch, J.Z. Zhao, M. Chen, E.D. Earle, and J. Cao. 2008. Suppression of diamondback moth using Bt-transgenic plants as a trap crop. Crop Protection 27(3–5):403–409.
Shields, E.J., J.T. Dauer, M.J. VanGessel, and G. Neumann. 2006. Horseweed (Conyza canadensis) seed collected in the planetary boundary layer. Weed Science 54(6):1063–1067.
Shipitalo, M.J., and L.B. Owens. 2006. Tillage system, application rate, and extreme event effects on herbicide losses in surface runoff. Journal of Environmental Quality 35(6):2186–2194.
Shipitalo, M.J., R.W. Malone, and L.B. Owens. 2008. Impact of glyphosate-tolerant soybean and glufosinate-tolerant corn production on herbicide losses in surface runoff. Journal of Environmental Quality 37(2):401–408.
Showalter, A.M., S. Heuberger, B.E. Tabashnik, and Y. Carrière. 2009. A primer for the use of insecticidal transgenic cotton in developing countries. Journal of Insect Science 9(22):1–39.
Siegfried, B.D., A.C. Zoerb, and T. Spencer. 2001. Development of European corn borer larvae on Event 176 Bt corn: Influence on survival and fitness. Entomologia Experimentalis et Applicata 100(1):15–20.
Simard, M.-J., A. Légère, and S.I. Warwick. 2006. Transgenic Brassica napus fields and Brassica rapa weeds in Quebec: Sympatry and weed-crop in situ hybridization. Canadian Journal of Botany 84(12):1842–1851.
Sisterson, M.S., L. Antilla, Y. Carrière, C. Ellers-Kirk, and B.E. Tabashnik. 2004a. Effects of insect population size on evolution of resistance to transgenic crops. Journal of Economic Entomology 97(4):1413–1424.
Sisterson, M.S., R.W. Biggs, C. Olson, Y. Carrière, T.J. Dennehy, and B.E. Tabashnik. 2004b. Arthropod abundance and diversity in Bt and non-Bt cotton fields. Environmental Entomology 33(4):921–929.
Sisterson, M.S., R.W. Biggs, N.M. Manhardt, Y. Carrière, T.J. Dennehy, and B.E. Tabashnik. 2007. Effects of transgenic Bt cotton on insecticide use and abundance of two generalist predators. Entomologia Experimentalis et Applicata 124(3):305–311.
Snow, A.A. 2002. Transgenic crops—why gene flow matters. Nature Biotechnology 20(6):542.
Snow, A.A., and P. Palma. 1997. Commercialization of transgenic plants: Potential ecological risks. BioScience 47(2):86–96.
Spencer, L.J., and A.A. Snow. 2001. Fecundity of transgenic wild-crop hybrids of Cucurbita pepo (Cucurbitaceae): Implications for crop-to-wild gene flow. Heredity 86(6):694–702.
Stewart, C.N., Jr., M.D. Halfhill, and S.I. Warwick. 2003. Transgene introgression from genetically modified crops to their wild relatives. Nature Reviews Genetics 4(10):806–817.
Storer, N.P., S.L. Peck, F. Gould, J.W. Van Duyn, and G.G. Kennedy. 2003. Spatial processes in the evolution of resistance in Helicoverpa zea (Lepidoptera: Noctuidae) to Bt transgenic corn and cotton in a mixed agroecosystem: A biology-rich stochastic simulation model. Journal of Economic Entomology 96(1):156–172.
Storer, N.P., G.P. Dively, and R.A. Herman. 2008. Landscape effects of insect-resistant genetically modified crops. In Integration of insect-resistant genetically modified crops within IPM programs. Vol. 5. eds. J. Romeis, A.M. Shelton, and G.G. Kennedy, pp. 273–302. New York: Springer Science + Business Media.
Stubbendieck, J.L., G.Y. Friisoe, and M.R. Bolick. 1994. Weeds of Nebraska and the Great Plains. Lincoln: Nebraska Department of Agriculture, Bureau of Plant Industry in cooperation with the University of Nebraska-Lincoln.
Tabashnik, B.E., and Y. Carrière. 2008. Evolution of insect resistance to transgenic plants. In Specialization, speciation, and radiation: The evolutionary biology of herbivorous insects. ed. K.J. Tilmon, pp. 267–279. Berkeley: University of California Press.
Tabashnik, B.E., and Y. Carrière. 2009. Insect resistant GM crops; pest resistance. In Environmental impact of genetically modified crops. eds. N. Ferry and A.M.R. Gatehouse, pp. 74–100. Wallingford, UK: CAB International.
Tabashnik, B.E., N. Finson, M.W. Johnson, and W.J. Moar. 1993. Resistance to toxins from Bacillus thuringiensis subsp. kurstaki causes minimal cross-resistance to B. thuringiensis subsp. aizawai in the diamondback moth (Lepidoptera: Plutellidae). Applied and Environmental Microbiology 59(5):1332–1335.
Tabashnik, B.E., A.L. Patin, T.J. Dennehy, Y.B. Liu, E. Miller, and R.T. Staten. 1999. Dispersal of pink bollworm (Lepidoptera: Gelechiidae) males in transgenic cotton that produces a Bacillus thuringiensis toxin. Journal of Economic Entomology 92(4):772–780.
Tabashnik, B.E., A.L. Patin, T.J. Dennehy, Y.B. Liu, Y. Carrière, M.A. Sims, and L. Antilla. 2000. Frequency of resistance to Bacillus thuringiensis in field populations of pink bollworm. Proceedings of the National Academy of Sciences of the United States of America 97(24):12980–12984.
Tabashnik, B.E., F. Gould, and Y. Carrière. 2004. Delaying evolution of insect resistance to transgenic crops by decreasing dominance and heritability. Journal of Evolutionary Biology 17(4):904–912.
Tabashnik, B.E., T.J. Dennehy, and Y. Carrière. 2005. Delayed resistance to transgenic cotton in pink bollworm. Proceedings of the National Academy of Sciences of the United States of America 102(43):15389–15393.
Tabashnik, B.E., J.A. Fabrick, S. Henderson, R.W. Biggs, C.M. Yafuso, M.E. Nyboer, N.M. Manhardt, L.A. Coughlin, J. Sollome, Y. Carrière, T.J. Dennehy, and S. Morin. 2006. DNA screening reveals pink bollworm resistance to Bt cotton remains rare after a decade of exposure. Journal of Economic Entomology 99(5):1525–1530.
Tabashnik, B.E., A.J. Gassmann, D.W. Crowder, and Y. Carrière. 2008. Insect resistance to Bt crops: Evidence versus theory. Nature Biotechnology 26(2):199–202.
———. 2008. Field-evolved resistance to Bt toxins. Nature Biotechnology 26(10):1074–1076.
Tabashnik, B.E., J.B.J. Van Rensburg, and Y. Carrière. 2009a. Field-evolved insect resistance to Bt crops: Definition, theory, and data. Journal of Economic Entomology 102:2011–2025.
Tabashnik, B.E., G.C. Unnithan, L. Masson, D.W. Crowder, X. Li, and Y. Carrière. 2009b. Asymmetrical cross-resistance between Bacillus thuringiensis toxins Cry1Ac and Cry2Ab in pink bollworm. Proceedings of the National Academy of Sciences of the United States of America 106(29):11889–11894.
Tarkalson, D.D., S.D. Kachman, J.M.N. Knops, J.E. Thies, and C.S. Wortmann. 2008. Decomposition of Bt and non-Bt corn hybrid residues in the field. Nutrient Cycling in Agroecosystems 80(3):211–222.
Terán-Vargas, A.P., J.C. Rodríguez, C.A. Blanco, J.L. Martínez-Carrillo, J. Cibrián-Tovar, H. Sánchez-Arroyo, L.A. Rodríguez-del-Bosque, and D. Stanley. 2005. Bollgard cotton and resistance of tobacco budworm (Lepidoptera: Noctuidae) to conventional insecticides in Southern Tamaulipas, Mexico. Journal of Economic Entomology 98(6):2203–2209.
Thompson, G.D., S. Matten, I. Denholm, M.E. Whalon, and P. Leonard. 2008. The politics of resistance management: Working towards pesticide resistance mangement globally. In Global pesticide resistance in arthropods. eds. M.E. Whalon, D. Mota-Sanchez and R.M. Hollingworth, pp. 146–164. Wallingford, UK: CAB International.
Tsui, M.T.K., and L.M. Chu. 2003. Aquatic toxicity of glyphosate-based formulations: Comparison between different organisms and the effects of environmental factors. Chemosphere 52(7):1189–1197.
Tuesca, D., E. Puricelli, and J.C. Papa. 2001. A long-term study of weed flora shifts in different tillage systems. Weed Research 41(4):369–382.
Uri, N.D., J.D. Atwood, and J. Sanabria. 1999. The environmental benefits and costs of conservation tillage. Environmental Geology 38(2):111–125.
US-EPA (U.S. Environmental Protection Agency). 1998. The Environmental Protection Agency’s white paper on Bt plant-pesticide resistance management. January 14. Washington, DC. Available online at http://www.epa.gov/EPA-PEST/1998/January/Day-14/paper.pdf. Accessed May 4, 2009.
———. 2000. Great Lakes binational toxics strategy: The level I pesticides in the binational strategy. March 1. Section 3. Washington, DC. Available online at http://www.epa.gov/greatlakes/bns/pesticides/finalpestreport.html. Accessed April 13, 2009.
———. 2001. Biopesticides registration action document—Bacillus thuringiensis plant-incorporated protectants. October 16. Washington, DC. Available online at http://www.epa.gov/pesticides/biopesticides/pips/bt_brad.htm. Accessed June 13, 2009.
———. 2005. Biopesticides registration action document: Bacillus thuringiensis var. aizawai Cry1F and the genetic material (from the insert of plasmid PGMA281) necessary for its production in cotton and Bacillus thuringiensis var. kurstaki Cry1Ac and the genetic material (from the insert of plasmid PMYC3006) necessary for its production in cotton. Washington, DC. Available online at http://www.epa.gov/pesticides/biopesticides/ingredients/tech_docs/brad_006512-006513.pdf. Accessed April 20, 2009.
———. 2006a. A set of scientific issues being considered by the Environmental Protection Agency regarding: Evaluation of the resistance risks from using 100% Bollgard and Bollgard II cotton as part of a pink bollworm eradication program in the state of Arizona. October 24–26. SAP Minutes No 2007-01. Washington, DC. Available online at http://www.epa.gov/scipoly/sap/meetings/2006/october/october2006finalmeetingminutes.pdf. Accessed March 31, 2010.
———. 2006b. A set of scientific issues being considered by the Environmental Protection Agency regarding: Analysis of a natural refuge of non-cotton hosts for Monsanto’s Bollgard II cotton. September 8. SAP Minutes No 2006-03. Washington, DC. Available online at http://www.epa.gov/scipoly/sap/meetings/2006/june/june2006finalmeet-ingminutes.pdf. Accessed January 22, 2009.
———. 2007. Pesticide news story: EPA approves natural refuge for insect resistance management in Bollgard II cotton. Washington, DC. Available online at http://www.epa.gov/oppfead1/cb/csb_page/updates/2007/bollgard-cotton.htm. Accessed January 22, 2009.
———. 2008a. Insect resistance management fact sheet for Bacillus thuringiensis (Bt) cotton products. Washington, DC. Available online at http://www.epa.gov/opp00001/biopesticides/pips/bt_cotton_refuge_2006.htm. Accessed January 22, 2009.
———. 2008b. Insect resistance management fact sheet for Bacillus thuringiensis (Bt) corn products. Washington, DC. Available online at http://www.epa.gov/oppbppd1/biopesticides/pips/bt_corn_refuge_2006.htm. Accessed January 22, 2009.
USDA-APHIS (U.S. Department of Agriculture–Animal and Plant Health Inspection Service). 2008. Determination of non-regulated status for glyphosate-tolerant (GlyTol™)) cotton, Gossypium hirsutum, event GHB614. May 16. Petition 06-332-01p. Washington, DC. Available online at http://www.aphis.usda.gov/brs/aphisdocs/06_33201p_pea.pdf. Accessed March 31, 2010.
USDA-ERS (U.S. Department of Agriculture–Economic Research Service). 2009. Agricultural biotechnology: Adoption of biotechnology and its production impacts. Washington, DC. Available online at http://www.ers.usda.gov/Briefing/Biotechnology/chapter1.htm. Accessed October 1, 2009.
———. 2006. Agricultural resources and environmental indicators, 2006 edition. EIB-16. Washington, DC. Available online at http://www.ers.usda.gov/publications/arei/eib16/. Accessed February 17, 2009.
USDA-NASS (U.S. Department of Agriculture–National Agricultural Statistics Service). 2001. Acreage. June 29. Cr Pr 2-5 (6-01). Washington, DC. Available online at http://usda.mannlib.cornell.edu/usda/nass/Acre//2000s/2001/Acre-06-29-2001.pdf. Accessed April 14, 2009.
———. 2003. Acreage. June 30. Cr Pr 2-5 (6-03). Washington, DC. Available online at http://usda.mannlib.cornell.edu/usda/nass/Acre//2000s/2003/Acre-06-30-2003.pdf. Accessed April 14, 2009.
———. 2005. Acreage. June 30. Cr Pr 2-5 (6-05). Washington, DC. Available online at http://usda.mannlib.cornell.edu/usda/nass/Acre//2000s/2005/Acre-06-30-2005.pdf. Accessed April 14, 2009.
———. 2007. Acreage. June 29. Cr Pr 2-5 (6-07). Washington, DC. Available online at http://usda.mannlib.cornell.edu/usda/nass/Acre//2000s/2007/Acre-06-29-2007.pdf. Accessed April 14, 2009.
———. 2008. Acreage. June 30. Cr Pr 2-5 (6-08). Washington, DC. Available online at http://usda.mannlib.cornell.edu/usda/nass/Acre//2000s/2008/Acre-06-30-2008.pdf. Accessed March 31, 2010.
———. 2009a. Data and statistics: Quick stats. Washington, DC. Available online at http://www.nass.usda.gov/Data_and_Statistics/Quick_Stats/index.asp. Accessed June 22, 2009.
———. 2009b. Acreage. June 30. Cr Pr 2-5 (6-09).Washington, DC. Available online at http://usda.mannlib.cornell.edu/usda/current/Acre/Acre-06-30-2009.pdf. Accessed November 24, 2009.
Van Deynze, A.E., F.J. Sundstrom, and K.J. Bradford. 2005. Pollen-mediated gene flow in California cotton depends on pollinator activity. Crop Science 45(4):1565–1570.
van Frankenhuyzen, K., and C. Nystrom. 2002. The Bacillus thuringiensis toxin specificity database. The Great Lakes Forestry Centre. Available online at http://www.glfc.forestry.ca/bacillus/. Accessed April 27, 2009.
van Rensburg, J.B.J. 2007. First report of field resistance by the stem borer, Busseola fusca (Fuller) to Bt-transgenic maize. South African Journal of Plant and Soil 24(3):147–151.
VanGessel, M.J. 2001. Glyphosate-resistant horseweed from Delaware. Weed Science 49:703–705.
Vercesi, M.L., P.H. Krogh, and M. Holmstrup. 2006. Can Bacillus thuringiensis (Bt) corn residues and Bt-corn plants affect life-history traits in the earthworm Aporrectodea caliginosa? Applied Soil Ecology 32(2):180–187.
Walker, K., M. Mendelsohn, S. Matten, M. Alphin, and D. Ave. 2003a. The role of microbial Bt products in U.S. crop protection. Journal of New Seeds 5(1):31–51.
Walker, K.R., M.D. Ricciardone, and J. Jensen. 2003b. Developing an international consensus on DDT: A balance of environmental protection and disease control. International Journal of Hygiene and Environmental Health 206(4-5):423–435.
Walklate, P.J., J.C.R. Hunt, H.L. Higson, and J.B. Sweet. 2004. A model of pollen-mediated gene flow for oilseed rape. Proceedings of the Royal Society B: Biological Sciences 271(1538):441–449.
Wang, P., J.Z. Zhao, A. Rodrigo-Simón, W. Kain, A.F. Janmaat, A.M. Shelton, J. Ferré, and J. Myers. 2007. Mechanism of resistance to Bacillus thuringiensis toxin Cry1Ac in a greenhouse population of the cabbage looper, Trichoplusia ni. Applied and Environmental Microbiology 73(4):1199–1207.
Wardle, D.A. 1995. Impacts of disturbance on detritus food webs in agro-ecosystems of contrasting tillage and weed management practices. Advances in Ecological Research 26:105–185.
Warwick, S.I., M.J. Simard, A. Légère, H.J. Beckie, L. Braun, B. Zhu, P. Mason, G. Séguin-Swartz, and C.N. Stewart Jr. 2003. Hybridization between transgenic Brassica napus L. and its wild relatives: Brassica rapa L., Raphanus raphanistrum L., Sinapis arvensis L., and Erucastrum gallicum (Willd.) O.E. Schulz. Theoretical and Applied Genetics 107(3):528–539.
Warwick, S.I., A. Légère, M.J. Simard, and T. James. 2008. Do escaped transgenes persist in nature? The case of an herbicide resistance transgene in a weedy Brassica rapa population. Molecular Ecology 17(5):1387–1395.
Warwick, S.I., A. Francis, and J. La Fleche, eds. 2000. Guide to wild germplasm of Brassica and allied crops (tribe Brassiceae, Brassicaceae). 2nd ed. Agriculture and Agri-Food Canada. Ottawa, Ontario, Canada: AAFC-ECORC.
Wauchope, R.D., T.M. Buttler, A.G. Hornsby, P.W.M. Augustijn-Beckers, and J.P. Burt. 1992. The SCS/ARS/CES pesticide properties database for environmental decision-making. Reviews of Environmental Contamination and Toxicology 123:1–156.
Wauchope, R.D., T.L. Estes, R. Allen, J.L. Baker, A.G. Hornsby, R.L. Jones, R.P. Richards, and D.I. Gustafson. 2002. Predicted impact of transgenic, herbicide-tolerant corn on drinking water quality in vulnerable watersheds of the mid-western USA. Pest Management Science 58(2):146–160.
Weaver, M.A., L.J. Krutz, R.M. Zablotowicz, and K.N. Reddy. 2007. Effects of glyphosate on soil microbial communities and its mineralization in a Mississippi soil. Pest Management Science 63(4):388–393.
Weise, E. 2007. Effects of genetically engineered alfalfa cultivate a debate. USA Today, February 15. p. 10D, Life section. Available online at http://www.usatoday.com/news/health/2007-02-14-alfalfa_x.htm. Accessed June 4, 2009.
Werth, J.A., C. Preston, I.N. Taylor, G.W. Charles, G.N. Roberts, and J. Baker. 2008. Managing the risk of glyphosate resistance in Australian glyphosate-resistant cotton production systems. Pest Management Science 64(4):417–421.
Westgate, M.E., J. Lizaso, and W. Batchelor. 2003. Quantitative relationships between pollen shed density and grain yield in maize. Crop Science 43(3):934–942.
WHO (World Health Organization). 2005. Glyphosate and AMPA in drinking-water. Geneva: World Health Organization. Available online at http://www.who.int/water_sanitation_health/dwq/chemicals/glyphosate/en/. Accessed March 11, 2009.
Williams, M.R. 2009. Cotton insect losses—2008. Paper presented at the Beltwide Cotton Conference (San Antonio, TX, January 5–8, 2008). National Cotton Council of America. Available online at http://ncc.confex.com/ncc/2009/webprogram/Paper9858.html. Accessed March 25, 2009.
Wilson, R.G., S.D. Miller, P. Westra, A.R. Kniss, P.W. Stahlman, G.W. Wicks, and S.D. Kachman. 2007. Glyphosate-induced weed shifts in glyphosate-resistant corn or a rotation of glyphosate-resistant corn, sugarbeet, and spring wheat. Weed Technology 21(4):900–909.
Wolfenbarger, L.L., S. Naranjo, J. Lundgren, R. Bitzer, and L. Watrud. 2008. Bt crop effects on functional guilds of non-target arthropods: A meta-analysis. PLoS ONE 3(5).
Wu, K. 2007. Monitoring and management strategy for Helicoverpa armigera resistance to Bt cotton in China. Journal of Invertebrate Pathology 95(3):220–223.
Wu, K., W. Li, H. Feng, and Y. Guo. 2002. Seasonal abundance of the mirids, Lygus lucorum and Adelphocoris spp. (Hemiptera: Miridae) on Bt cotton in northern China. Crop Protection 21(10):997–1002.
Wu, K., W. Mu, G. Liang, and Y. Guo. 2005. Regional reversion of insecticide resistance in Helicoverpa armigera (Lepidoptera: Noctuidae) is associated with the use of Bt cotton in northern China. Pest Management Science 61(5):491–498.
Wu, K.-M., Y.-H. Lu, H.-Q. Feng, Y.-Y. Jiang, and J.-Z. Zhao. 2008. Suppression of cotton bollworm in multiple crops in China in areas with Bt toxin-containing cotton. Science 321(5896):1676–1678.
Xanthopoulos, F.P., and U.E. Kechagia. 2000. Natural crossing in cotton (Gossypium hirsutum L.). Australian Journal of Agricultural Research 51(8):979–983.
Yang, Y., H. Chen, Y. Wu, Y. Yang, and S. Wu. 2007. Mutated cadherin alleles from a field population of Helicoverpa armigera confer resistance to Bacillus thuringiensis toxin Cry1Ac. Applied Environmental Microbiology 73(21):6939–6944.
Yenish, J.P., D.A. Ball, and R. Schirman. 2009. Integrated management of jointed goatgrass in the Pacific Northwest. EB2042. Washington State University. Pullman, WA. Available online at http://cru.cahe.wsu.edu/CEPublications/EB2042/eb2042.pdf. Accessed November 18, 2009.
York, A.C., J.B. Beam, and A.S. Culpepper. 2005. Weed science: Control of volunteer glyphosate-resistant soybean in cotton. Journal of Cotton Science 9(2):102–109.
Zeimen, M.B., K.A. Janssen, D.W. Sweeney, G.M. Pierzynski, K.R. Mankin, D.L. Devlin, D.L. Regehr, M.R. Langemeier, and K.A. McVay. 2006. Combining management practices to reduce sediment, nutrients, and herbicides in runoff. Journal of Soil and Water Conservation 61(5):258–267.
Zelaya, I.A., and M.D.K. Owen. 2000. Differential response of common waterhemp (Amaranthus rudis) to glyphosate in Iowa. In Proceedings of the Weed Science Society of America, pp. 62–63 (Toronto, Canada). Weed Science Society of America.
Zelaya, I.A., and M.D.K. Owen. 2002. Amaranthus tuberculatus (Mq. ex DC) J.D. Sauer: Potential for selection of glyphosate resistance. In Proceedings of the 13th Australian Weeds Conference, Vol. 13, pp. 630–633 (Perth, Australia). Council of Australian Weed Science Societies.
Zelaya, I.A., M.D.K. Owen, and M.J. VanGessel. 2004. Inheritance of evolved glyphosate resistance in horseweed (Conyza canadensis (l.) Cronq.). Theoretical Applied Genetics 110:58–70.
———. 2007. Transfer of glyphosate resistance: Evidence of hybridization in Conyza (Asteraceae). American Journal of Botany 94(4):660–673.
Zhang, B.H., X.P. Pan, T.L. Guo, Q.L. Wang, and T.A. Anderson. 2005. Measuring gene flow in the cultivation of transgenic cotton (Gossypium hirsutum L.). Molecular Biotechnology 31(1):11–20.
Zhang, W., T.H. Ricketts, C. Kremen, K. Carney, and S.M. Swinton. 2007. Ecosystem services and dis-services to agriculture. Ecological Economics 64(2):253–260.
Zhao, J.Z., J. Cao, H.L. Collins, S.L. Bates, R.T. Roush, E.D. Earle, and A.M. Shelton. 2005. Concurrent use of transgenic plants expressing a single and two Bacillus thuringiensis genes speeds insect adaptation to pyramided plants. Proceedings of the National Academy of Sciences of the United States of America 102(24):8426–8430.
Zwahlen, C., W. Nentwig, F. Bigler, and A. Hilbeck. 2000. Tritrophic interactions of transgenic Bacillus thuringiensis corn, Anaphothrips obscurus (Thysanoptera: Thripidae), and the predator Orius majusculus (Heteroptera: Anthocoridae). Environmental Entomology 29(4):846–850.
Zwahlen, C., A. Hilbeck, R. Howald, and W. Nentwig. 2003. Effects of transgenic Bt corn litter on the earthworm Lumbricus terrestris. Molecular Ecology 12(4):1077–1086.
Zwahlen, C., A. Hilbeck, and W. Nentwig. 2007. Field decomposition of transgenic Bt maize residue and the impact on non-target soil invertebrates. Plant and Soil 300(1–2):245–257.