Appendix J
Pathogenicity Acquisition
The following sections discuss mechanisms, and provide examples, of how a microorganism may acquire pathogenicity.
GENE GAIN
Generation of Multidrug Resistant Bacterial Pathogens
The emergence of multidrug resistant bacterial pathogens, including Staphylococcus aureus, Mycobacterium tuberculosis, and Pseudomonas aeruginosa, highlights the importance of understanding the mechanisms by which antibiotic resistance is transferred among different genera of bacteria. The acquisition of resistance to multiple antimicrobial agents is a hallmark of methicillin-resistant S. aureus (MRSA), organisms that can cause life-threatening skin and soft tissue infections, endocarditis, and pneumonia. The SCCmec cassette, which confers resistance to methicillin and other β-lactams, is chromosomally-encoded but is transferred by a mobile genetic element. Mobile genetic elements, including transposons, plasmids, and bacteriophage, are the most common sources of acquired antibiotic resistance in MRSA. In addition, spontaneous mutations in chromosomally encoded genes can confer resistance to antibiotics (e.g., rpsL and streptomycin).
The two types of MRSA circulating within the human population are community-acquired (CA-MRSA) and healthcare-associated (HA-MRSA) MRSA. HA-MRSA strains have been propagated in healthcare facilities in the face of antibiotic pressure and continue to survive and multiply as a result of acquisition of multiple elements conferring resistance to antibiotics. In contrast, CA-MRSA strains have been propagated in the absence of extreme selective
pressure and have acquired resistance to fewer antibiotics. Both types of MRSA maintain chromosomal mutations that confer resistance to some therapies; these mutations are selected for and maintained in healthcare environments. HA-MRSA strains cause disease in immunocompromised individuals and are rarely isolated from patients with MRSA infections that are not linked to healthcare settings. In contrast, CA-MRSA strains typically cause infection in immunocompetent and otherwise healthy people. The mechanisms underlying this difference are still unclear and are likely multifactorial. Some evidence suggests that the acquisition of multiple antibiotic-resistance determinants by HA-MRSA strains actually reduces the “fitness” of the organism, which makes them unable to colonize and/or infect otherwise healthy people.
Toxins
Protein toxins in bacteria are often associated with mobile genetic elements such as phages and plasmid DNA. For this reason many toxin genes have spread between species by horizontal gene transfer. Production of active toxin sometimes requires the presence of accessory proteins for posttranslational modification and/or export. Thus the detection of a toxin gene is not necessarily predictive of virulence. These toxins may be grouped into two broad categories, structural and enzymatic. Structural toxins produce an effect solely through interactions with the target cell while enzymatic toxins catalyze a specific reaction that has an effect on the cell.
Acquisition of Shiga toxin-encoding bacteriophage. The emergence of Shiga toxin-producing E. coli strains in the early 1980s provides a clear example of how common bacteria may acquire bacteriophages that encode virulence traits such as bacterial toxin genes. This horizontal transfer of genetic material among bacteria via phage infection can result in the rapid evolution of new pathogens. The E. coli serotype O157 cited above was previously unknown as a pathogen in humans; however, the incorporation of genes homologous to those that encode Shiga toxin in the related agent of bacillary dysentery, Shigella dysenteriae type 1, generated a strain that produces hemorrhagic colitis and the life-threatening hemolytic uremic syndrome in humans.
Shiga toxins are AB5 toxins (one polypeptide chain that has enzymatic activity and 5 cell-binding subunits) that are among the most potent toxins known. They kill sensitive cells by shutting down protein synthesis in a manner identical to that of ricin. Indeed, Shiga toxins, like ricin, are classified as SAs. In addition to E. coli O157, several other serotypes of E. coli and some related species including Shigella sonnei, Aeromonas hydrophila, and Enterobacter cloacae have been described that encode related Shiga-type toxins within lysogenic (chromosomally-integrated) bacteriophages and cause disease in humans. Bacteriophages are thought to be the most plentiful infectious forms on Earth.
The ubiquitous nature of these agents, and the frequency with which they recombine with one another during bacterial infection, provides an enormous opportunity for the exchange of phage and bacterial DNA. Furthermore, during the lytic cycle of bacteriophage replication, numerous phage particles are generated and released upon lysis of an infected bacterium. This provides the opportunity for additional bacterial infections and transfer of phage-encoded virulence factors. Indeed, transfer of Shiga toxin-bearing phages from pathogenic strains of E. coli to “bystander” E. coli strains that constitute normal intestinal flora has been observed during experimental infections in animals and certainly occurs in nature.
Cholera toxin acquisition via toxin-coregulated pilus mediated CTXΦ uptake. Vibrio cholerae is the etiological agent of cholera, a disease that manifests as either mild self-limited diarrhea or potentially fatal watery diarrhea and vomiting. The hallmark watery diarrhea results from intoxication with cholera toxin (CT), an AB5 toxin that binds to the ganglioside GM1 on the surface of intestinal epithelial cells. Five B subunits form a pentameric pore to facilitate entry of the A subunit into the cell; the internalized A subunit transfers ADP-ribose to a G protein that activates adenylate cyclase, which leads to increased cAMP production and hypersecretion of water and electrolytes. The genes encoding CT (ctxAB) are encoded on CTXΦ, a filamentous phage that is thought to be transferred from one bacterium to another during infection of the small intestine (McLeod, Kimsey et al. 2005). The V. cholerae surface receptor for CTXΦ is the toxin-coregulated pilus (TCP), a type IV bundle-forming pilus whose synthesis is encoded by genes found on a PAI on the V. cholerae large chromosome. In addition to its function as the receptor for CT, TCP is factor that is required for V. cholerae intestinal colonization. Once CTXΦ is internalized into the bacterial cell, it integrates into the host genome using host machinery. Transcription of phage genes required for phage replication and virion production is repressed during lysogeny. Expression of ctxAB and the tcp loci is controlled by a complex network of regulatory elements known as the ToxR regulon (Matson, Withey et al. 2007). Unlike most phage that encode virulence factors, CTXΦ is never excised from the chromosome; the mechanism underlying this phenomenon is not known. Instead, phage replication occurs during genome replication, and new virions are produced as a result of transcription and translation of integrated chromosomal phage genes when transcription is derepressed under stress conditions (McLeod, Kimsey et al. 2005). Virions are secreted using machinery encoded by both the phage and the host; once secreted into the extracellular milieu, phage can bind to the TCP of a susceptible host cell and be internalized. Identification of the ctxAB genes in the Vibrio cholerae genome would suggest that the particular strain could cause water diarrhea; however, regulation of ctxA is sufficiently complicated that mere identification of the toxin genes would not be predictive of virulence.
Capsule Switching in Streptococcus Pneumoniae
Streptococcus pneumoniae is an etiologic agent of otitis media, pneumonia, meningitis, and septicemia. The incidence of invasive pneumococcal disease has decreased since the introduction of two vaccines that protect against infection with S. pneumoniae. The vaccines are composed of capsular polysaccharides from 7 (Prevnar™) or 23 (Pneumova®) of the most commonly isolated serotypes of S. pneumoniae. To date, 91 serotypes of S. pneumoniae have been described based upon their unique capsule structures (Park, Pritchard et al. 2007). Recombination of capsule loci, or capsular switching, is a common mechanism utilized by S. pneumoniae to evade host defenses. The regions flanking the capsule loci are very similar among capsular serotypes, and this sequence characteristic facilitates homologous recombination of capsule genes acquired through horizontal gene transfer in this naturally competent organism. Routine vaccination of young children with Prevnar™ has led to a significant decrease in the incidence of pneumococcal disease caused by the 7 serotypes in the vaccine. However, because S. pneumoniae is readily able to undergo horizontal transfer of its capsule genes, non-vaccine serotypes of the pneumococcus have emerged as important causative agents of invasive disease. Thus, the bacteria are rapidly adapting to an alteration in host susceptibility.
O-Antigen Evolution in Salmonella
Salmonella species are masters of adaptation to the host environment (Kingsley and Bäumler 2000). Upon infection with a Salmonella species to which it is susceptible, the host will develop symptoms of salmonellosis, which, in humans, is often characterized by gastroenteritis. The majority of humans will resolve the symptoms of Salmonella typhimurium subsp typhi infection within a day or two. In a small subset of cases, the bacteria enter the bloodstream to cause bacteremia. Ultimately, Salmonella migrates to the gall bladder, which is an immunologically protected environment, and resides there for years in a chronic colonization state. Occasionally Salmonella are released back into the intestine when bile is secreted, which allows for shedding of the organism and transmission to a new host.
The vast majority of mammals that become infected with Salmonella spp. produce antibodies against the lipopolysaccharide (LPS) O-antigen; O-antigen is the highly variable terminal oligosaccharide structure that forms the basis for the serogroups of Salmonella. Once a host develops antibodies against the O-antigen, the host is protected from reinfection with the same or a different strain that harbors the same O-antigen. Within a population, infection followed by protective antibody generation eventually reduces the virulence of strains that harbor a particular O-antigen to the point that the organism must evolve in order to survive. The genes that encode the enzymes required for O-antigen
synthesis routinely undergo horizontal gene transfer in the face of host adaptation. When the O-antigen structure changes, the population becomes susceptible to infection with the strain that expresses the newly acquired O-antigen, and the cycle begins anew.
GENE LOSS
Shigella spp
The evolution of a non-pathogenic bacterial species into a genetically related but pathogenic species typically occurs as a result of the acquisition of genes encoding virulence factors, often via transfer of pathogenicity islands. However, loss of a gene or genes can also be critical to the capacity of a non-pathogenic organism to be converted to one that causes serious disease. Such is the case for the evolution of non-pathogenic Escherichia coli into pathogenic Shigella spp. and the genetically and clinically similar enteroinvasive E. coli (EIEC). Laboratory strains of E. coli contain a gene, cadA, that encodes lysine decarboxylase, an enzyme that catalyzes the decarboxylation of lysine to form cadaverine. The cadA locus has been deleted from E. coli during the evolution of the pathogenic Shigella spp. and EIEC. While cadaverine does not affect the invasive capacity of Shigella spp. and EIEC, it completely inhibits enterotoxin activity of Shigella spp.(Maurelli, Fernandez et al. 1998). Thus, the loss of cadA enhanced the virulence of the Shigella spp. and EIEC.
Y. pestis
Gene loss was a key mechanism in the evolution of Yersinia pseudotuberculosis to Y. pestis. Y. pseudotuberculosis is a free-living bacterium that causes mild gastroenteritis in humans and animals. In contrast, Y. pestis is an extremely virulent organism that causes bubonic and pneumonic plague. The host range for the two organisms differs considerably in that Y. pestis requires a vector (the flea) for transmission between mammalian hosts, while Y. pseudotuberculosis is transmitted freely between mammalian hosts. Genomic analyses of representative strains from each species demonstrated that, despite the difference in host range, the two organisms are very closely related at the nucleotide level, and that Y. pseudotuberculosis is the most recent ancestor of Y. pestis (Chain P). However, and perhaps owing to the need to adapt to different host niches, up to 13 percent of the Y. pestis chromosome is inactivated.
GENE MUTATION
Filoviruses. The Marburg and Ebola filoviruses and the SARS-CoV coronavirus replicate poorly in adult mice. Mouse adaptation of these viruses has
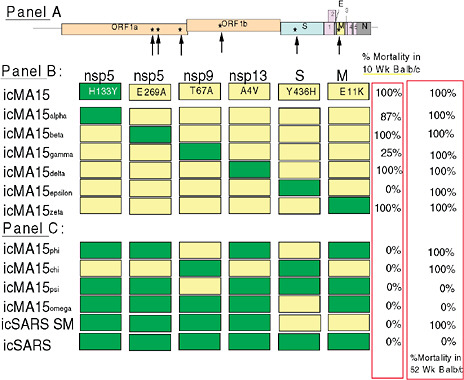
FIGURE J.1 Genome organization of icMA15 (Panel A). A series of chimeric icMA15 viruses were generated with different subsets of 5 icMA15 mutations in the icSARS-CoV WT genome, noting that wildtype positions in nsp9 and the S gene attenuated virulence in young but not aged animals (Panel B). Smaller combinations of the MA15 mutation set showed that 2-set nsp9/S or S/M were sufficient to kill aged animals only (Panel C).
provided an opportunity to identify mutation sets that are responsible for high virulence and cross species adaptation, although the approach is often limited by the isogenic host background as compared with outbred populations in natural settings. By blind serial passage in progressively older mice, mouse-adapted Ebola (MA-ZEBOV) acquired eight amino acid changes that were associated with high virulence, as well as a variety of silent changes. Using recombinant viruses, introduction of wild-type alleles into MA-ZEBOV VP24 and/or the NP protein were significantly associated with decreased virulence in the BALB/c mouse model. Moreover, efficient MA-ZEBOV replication in mice required the mouse adapted NP and VP24 genes, the latter is a potent inhibitor of type I interferon signaling. Recombinant viruses encoding the MA-VP24 mutation grew extremely efficiently in IFN-treated mouse peritoneal
macrophage cell lines. The data indicate that VP24 type I interferon antagonist activity is a significant virulence factor for ZEBOV replication and pathogenesis in mammals, although the molecular mechanisms governing its in vivo function remain unclear (Ebihara, Takada et al. 2006). Ebola VP24-mediated inhibition of cellular responses to IFNs correlates with the impaired nuclear accumulation of tyrosine-phosphorylated STAT1; mostly likely by VP24 interactions with karyopherin alpha1, but not with karyopherin alpha2, alpha3, or alpha4. It is possible that the mouse adapted mutations in VP24 confer increased targeting and antagonism of murine Karyopherin alpha 1 protein import machinery, although direct proof is lacking. Serial passage of Marburg virus was first done in immunodeficient (SCID) mice, and then in immunocompetent mice. In vivo adaptation has also resulted in a mouse-adapted strain MARV-Ravn. MARV-Ravn also caused uncontrolled viremia and high viral titers in the liver, spleen, lymph node, and other organs; profound lymphopenia; destruction of lymphocytes within the spleen and lymph nodes; and marked liver damage and thrombocytopenia in BALB/c mice. Sequence analysis of the mouse-adapted MARV-Ravn strain revealed differences in 16 predicted amino acids from the progenitor virus, although the exact changes required for adaptation are unclear at this time.
Serial passage of the late phase epidemic SARS-CoV Urbani in 10-week-old BALB/c mouse lungs also resulted in mouse adapted strains after 15 or 25 2-3 day passages. Under near identical conditions in 1-year-old animals, only 5 passages were sufficient for mouse adaptation demonstrating the increased susceptibility of aged populations to lethal SARS-CoV infection (data not shown). Importantly, these viruses cause an ARDS phenotype reminiscent of acute human infections in aged animals, and pneumonia in young animals. Thus, age, genetic background of the host and immune status likely facilitate cross-species transmission and adaptation potential of zoonotic RNA viruses. Sequence analysis of young-mouse-adapted strains revealed mutations in common genes sets, notably nsp5, nsp9, the M glycoprotein genes, including changes within the RBD of S. Unique targets in icMA15 and MA25 included nsp3, nsp12 and nsp13. Using recombinant chimeras between icMA15 and wildtype SARS-CoV, mutations in nsp9 and the S glycoprotein were most important for eliciting fatal disease in young, but not aged animals, although these changes were still highly interdependent on the other mutations as well. In aged animals, however, smaller subsets (2 mutations) of mutations encoded by icMA15 genotype were capable of producing fatal disease. Interdependency of the allele sets was mostly lost as virtually all two or four set combinations tested were lethal and the S gene mutation set also resulted in significant morbidity with an ARDS pathogenic phenotype. These data are consistent with the idea that aged populations are more compliant hosts for rapid SARS-CoV adaptation to virulence.
Although the mutation sets in S were different in icMA15 and MA25, both
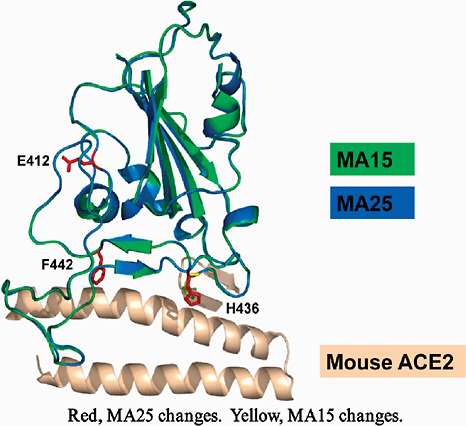
FIGURE J.2 Mouse adapted mutations in the S glycoprotein RBD-hACE interaction site.
sets of RBD mutation putatively enhanced S interaction with the mouse ACE2 receptor, likely increasing virus replication efficiency in the lung by 2-3 logs of titer (See above Figure. Mouse Adapted Mutations in the S Glycoprotein RBD-hACE Interaction Site). Other unique pathways to SARS-CoV S RBD mACE2 recognition have also been identified (data not shown), reiterating the plasticity of coronavirus RBD-receptor interaction networks. Thus, the in vivo models of SARS-CoV adaptation to the murine host reflected the key role that the S glycoprotein plays in mediating cross-species adaptation and pathogenesis. Notably, and in contrast to results seen with filoviruses, no evolution was noted in the six different antagonists of innate immunity. It is noteworthy that each in vivo adapation model selected for unique sets of mutations that enhanced virus replication efficiency, resulting in increased pathogenic outcomes. Although mutation sets targeted key genes essential for efficient virus-host interaction,
the actual mutation sets were different, demonstrating the existence of multiple genetic pathways to increased virulence and the same pulmonary disease outcome. Moreover, host background (e.g., genetics, age, immune status, etc.) was a major selective force in the evolution of virulence, further complicating the development of universal laws governing cross-species transmission and virulence.
GENE REGULATION
Regulation of Bacterial Pathogenicity
If an organism possesses specialized gene products for its virulence, it must be able to utilize them when needed, but not squander its metabolic energy producing them aimlessly or risk having them detected by host defenses and neutralized prematurely. Consequently, regulation of virulence factor expression is an additional, yet essential, complication of a pathogenic microorganism’s life. The host presents an array of conditions that are strikingly distinct from those of the outside environment, conditions that are not easily reproduced in the laboratory. In fact, laboratory culture conditions bias our understanding of microbial adaptation to natural environments. V. cholerae, for example, is thought to persist in brackish estuaries and other saline aquatic environments, sometimes associated with the chitinous exoskeleton of various marine organisms. Transition from this milieu to the contrasting environment of the human small intestinal lumen must be accompanied by substantial genetic regulatory events.
The microbial cell is relatively simple, yet it possesses the means to detect, often simultaneously, changes in temperature, ionic conditions, oxygen concentration, pH, and calcium, iron, and other metal concentrations that might appear to be subtle signals, but which are essential for the precise mobilization of virulence determinants. Similarly, environmental regulatory signals prepare the microorganism for its transition from an extracellular to an intracellular existence. For example, iron is a critical component of many cell metabolic processes; therefore, it is not surprising that animals rely on high-affinity iron-binding and storage proteins to deprive microorganisms from access to this nutrient, especially at the mucosal surface. In turn, most pathogens sense iron availability and induce or repress various iron acquisition systems accordingly. Indeed, many microorganisms possess toxins that are regulated by iron such that low iron concentrations trigger toxin biosynthesis. In addition, reversible regulation of virulence gene expression by temperature is a feature common to many pathogens. For example, E. coli may be deposited in feces and live for long periods of time under conditions of nutrient depletion and low temperature. However, it has learned to mobilize its colonization-specific genes when it is returned to the warm mammalian body. The regulatory machinery used to
accomplish this is an important feature of many pathogens, including Yersinia pestis and B. anthracis, both of which exist in multiple niches within and outside the mammalian host.
The number of well-characterized virulence regulatory systems is rapidly increasing, in part because of the development of rapid methods for screening gene expression on a genome-wide basis (e.g., with the use of DNA microarrays). At the same time, relatively little is known about both the specific environmental signals to which these systems respond and the exact role of these responses in the course of human infection. One common mechanism for bacterial transduction of environmental signals involves two-component regulatory systems that act on gene expression, usually at the transcriptional level. Such systems make use of similar pairs of proteins; one protein of the pair spans the cytoplasmic membrane, contains a transmitter domain, and may act as a sensor of environmental stimuli, whereas the other is a cytoplasmic protein (“response regulator”) with a receiver domain that regulates responsive genes or proteins. These regulatory systems are common in both pathogens and non-pathogens so their detection by sequence analysis cannot be employed as a reliable predictor of pathogen vs. non-pathogen.
The coordinated control of pathogenicity incorporates the important concept of a regulon. A regulon is a group of operons and/or individual genes controlled by a common regulator, usually a protein activator or repressor. A regulon provides a means by which many genes can respond in concert to a particular stimulus. At other times the same genes may respond independently to other signals. Global regulatory networks are a common feature of microbial virulence as well as basic microbial physiology and therefore their sequences, while often essential for a pathogen, are also not reliable predictors of virulence. The apparent complexity of virulence regulation in a single microbial pathogen is magnified by the coexistence of multiple interacting (“cross-talking”) systems and by regulons within regulons.
Proper presentation of certain virulence-associated gene products on the microbial surface is now recognized to be as important to pathogenicity as the initial expression of these genes. Presentation entails export pathways, association with other periplasmic or surface factors, macromolecular assembly at the surface (for some), as well as regulation of the export components themselves. Among bacterial pathogens and non-pathogens alike that inhabit the human body, shared homology is apparent among families of proteins involved in these processes. Folding, transport, and assembly of specific proteins enable a microorganism to present a specific array of surface molecules necessary for eukaryotic cell tropism, intoxication, or entry. The precise configuration of a number of microbial surface molecules might be viewed as a cooperative “attack complex,” a property not found in any of the individual components. However, remarkably similar complexes are used by harmless microorganisms in the colonization of mucosal surfaces. In one case, the harmless microorgan-
ism remains attached to the host surface and causes no harm while, in the other case, the organism attaches to the mucosal surface and breaches this barrier to cause infection and disease.
Pathogenic microorganisms have developed many different mechanisms by which to regulate expression of virulence factors. For organisms with multiple hosts, such as Y. pestis, differential expression of virulence factors is required for adaptation and survival within each host environment. In the case of commensal organisms that cause infection opportunistically, such as Staphylococcus aureus, control of virulence factor expression is niche-dependent.
The lifestyle of Y. pestis requires survival of the organism in the flea host and the mammalian host. The primary reservoir for Y. pestis is rodents. Rodent-to-rodent transmission can occur, which can lead to epizootic plague. Fleas become infected by feeding on rodents that are bacteremic as a result of Y. pestis infection. The bacteria multiply rapidly in the flea midgut, and cause blockage of the proventriculus. Transmission of Y. pestis from flea to human occurs as the flea bites and attempts to feed on the human. Because the proventriculus is blocked, the flea regurgitates the Y. pestis into the bite, which, in turn, may lead to human infection. The virulence factors required for each host environment are regulated primarily by the temperature of the host. LcrF and Caf1R are positive regulators of virulence factors that are expressed in the mammalian host (Reviewed by Konkel and Tilly 2000). LcrF regulates expression of ~50 genes that encode the Yops (Yersinia outer proteins); the Yop effector proteins are secreted via a type III secretion system that is part of the Yop family. In addition to being controlled by temperature, lcrF is regulated post-transcriptionally because the ribosome binding site of the lcrF transcript is sequestered in a stem-loop structure at low temperature. When the host temperature increases (i.e., when the bacteria enter the mammalian host), the stem-loop unfolds and permits binding of the ribosome to promote translation of lcrF. Caf1R regulates expression of the Y. pestis capsule genes. The capsule provides protection from phagocytosis of Y. pestis; such protection is critical to survival of the organism in the mammalian bloodstream. In contrast, the hms locus is induced at low temperature, when Y. pestis is in the flea. The hms locus encodes genes whose products are required for hemin storage. Inactivation of the hms genes results in Y. pestis strains that are unable to block the flea proventriculus, but, as anticipated, has no effect on pathogenesis in mammalian hosts because the genes are expressed only at low temperature (in the flea).
In conclusion, the inherent pathogenicity of a microorganism can be altered by gene additions or losses or through genetic regulation of essential genes for virulence.