2
Complexity of the Contaminated Subsurface
No environmental problem can be solved rationally before it has been adequately defined. This chapter presents an overview of the characteristics of contaminated ground water that must be understood in order to assess prospects for remediation. The complexities of the subsurface environment and of contaminant distribution, which are documented in this chapter, significantly complicate the cleanup task (see Box 2-1). Although this chapter focuses on factors that complicate ground water cleanup, the arguments should not be interpreted to mean that restoration of ground water is impossible on theoretical grounds. Ground water contamination by hazardous substances is by no means intractable, although at many sites it is likely to prove extraordinarily difficult, time consuming, and costly to reverse.
THE SUBSURFACE ENVIRONMENT
When contaminants enter the subsurface, they become subject to a variety of physical, chemical, and biological processes that operate beneath the ground. The design of an effective ground water cleanup system requires an understanding of these processes because they control the fate of the contaminants and the ease with which they can be extracted.
Physical Characteristics
Ground water is stored in underground formations called aquifers. There are two broad categories of aquifers: consolidated (see Figure 2-1)
BOX 2-1 COMPLEXITY OF THE CONTAMINATED SUBSURFACE: A HYPOTHETICAL EXAMPLE Every conceivable subsurface remediation approach is subject to constraints posed by fundamental principles such as conservation of mass, conservation of energy, the theoretical limitation on energy efficiency embodied in the Second Law of Thermodynamics, and the thermodynamic relationships governing chemical and physical equilibria. Nothing in these principles precludes cleanup of contaminated sites to any desired level. However, consideration of these laws makes it evident that cleanup using presently known technology will often be extremely difficult to achieve in a reasonable period of time. To illustrate the situation, consider a barrel of trichloroethylene (TCE) that has leaked below ground. Because the TCE disperses, it would not generally be possible to pump the chemical out in pure form; rather, the TCE must dissolve in the ground water and be removed by pumping out the water. Based on the solubility of TCE in water, more than one thousand barrels of water would have to be removed for each barrel of TCE spilled, if the water removed was saturated with TCE. In practice, however, the pumped water may contain only one-tenth or one-hundredth or one-thousandth of the amount of TCE that would be present if the water were completely saturated with TCE, because most of the water will never have been in dose enough contact to the TCE long enough to allow complete saturation. Then, for each barrel of TCE it will be necessary to pump out 10,000 or 100,000 or 1 million barrels of water. Wells placed near the TCE would speed up the process, but it is seldom possible to precisely locate the concentrated pockets of chemical contamination. This example illustrates common elements of ground water contamination problems that greatly interfere with cleanup efforts; contaminants may be difficult to extract because they do not dissolve fully; the subsurface environment is neither simple in structure nor easy to characterize, and determining the precise location of the contamination is seldom easy. |
and unconsolidated (see Figure 2-2). Unconsolidated aquifers consist of uncemented granular materials such as sand and gravel; they store water in the interstitial pore space among the grains. Consolidated aquifers consist of more or less solid rock; they store water primarily in solution channels, fractures, and joints (although in material such as sandstone, some water may also be stored in interstitial pore spaces). Layers of such formations comprise what is called the saturated ground water zone. Here, water completely fills the pore openings. Overlying the saturated zone is a zone in which the pore spaces contain both air and water and thus are not saturated with water. This zone is known as the unsaturated, or vadose, zone.
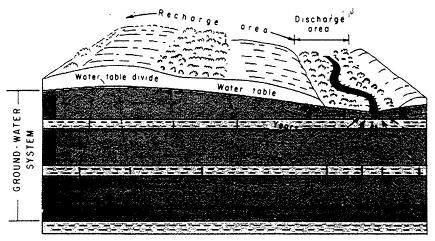
FIGURE 2-1 Simplified schematic of ground water flow in an unconsolidated aquifer. The flow lines indicate travel times to various parts of the subsurface, with longer travel times indicated by flow lines reaching deeper into the subsurface. SOURCE: Heath (1983).
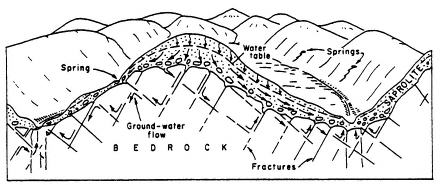
FIGURE 2-2 Simplified schematic of ground water flow in a consolidated aquifer. As the flow lines indicate, the direction of ground water flow in such aquifers depends on the locations of the fractures and thus is often tortuous and difficult to predict. SOURCE: From Heath (1980), as reprinted in LeGrand (1988).
Hydraulic Properties of Aquifers
Table 2-1 summarizes key hydraulic properties of aquifers and their importance to remediation efforts. (See the glossary for definitions of hydraulic properties and other technical terms used in this chapter.) As
TABLE 2-1 Hydraulic Properties of Aquifers Important for Ground Water Cleanup
Property |
Description |
Importance for Ground Water Cleanup |
Porosity |
Volume of pore space relative to the total volume |
Pores store water and contaminants |
Effective porosity |
Interconnected pore space that can transmit fluid |
Water and contaminants flow through interconnected pores |
Ground water velocity |
Rate of fluid movement |
Influences the direction and velocity of dissolved contaminant movement |
Hydraulic gradient |
Elevation and pressure differences that cause fluids to flow |
Influences the direction of contaminant movement |
Hydraulic conductivity |
Ease with which water can move through a formation |
Influences the rate at which fluid can be pumped for treatment |
Transmissivity |
Product of formation thickness and hydraulic conductivity |
Influences the rate at which fluid can be pumped for treatment |
Storage coefficient |
Volume released by pressure changes per unit area during pumping in a confined aquifer |
Influences the quantity of fluid that can be obtained by pumping |
Specific yield |
Fraction of total pore volume released as water by gravity drainage during pumping of an unconfined aquifer |
Influences the quantity of fluid that can be obtained by pumping |
Specific retention |
Fraction of total aquifer volume retained as water above the water table after pumping an unconfined formation |
Influences the quantity of contaminant that remains in the subsurface after pumping |
an illustration of why hydraulic properties are important in ground water remediation, consider the effect of variations in hydraulic conductivity. The hydraulic conductivity controls the amount of water that can be supplied to a well and therefore reflects the ease with which dissolved contaminants can be removed from the aquifer. The hydraulic conductivity of a sandy aquifer is approximately two orders of magnitude greater than the hydraulic conductivity of a silty sand aquifer (average hydraulic conductivities for these types of materials are 30 meters per day and 0.3 meters per day, respectively). Accordingly, water containing contaminants can be extracted from a sandy aquifer at a rate about 100 times greater than it can be extracted from a silty sand aquifer. Therefore, if the zone of contamination is the same in both aquifers, it will be
possible to clean up the sandy aquifer much faster than the silty sand aquifer. However, the ease of cleanup of the clean sand aquifer relative to the silty sand aquifer may be offset by the fact that the zone of contamination will be larger in the clean sand aquifer because it is more permeable. This example illustrates that understanding the aquifer's key hydraulic properties and how they are likely to influence the remediation is essential to predicting the performance of the cleanup system.
Determining the influence of hydraulic properties on remediation is far more difficult in fractured, consolidated rocks than in unconsolidated rocks because the hydraulic properties vary widely with location and therefore depend on the size of the sample of the aquifer being investigated. For example, a small volume of rock obtained from between fractures can have an exceedingly low porosity; on the other hand, a small part of aquifer material primarily from a fracture can have a porosity approaching 100 percent. Therefore, the true values for a reasonably sized portion of the aquifer are between these two extremes. An average value of the hydraulic parameters for such an aquifer is of little use in providing hydrogeologic information required for cleaning up aquifers. Predicting contaminant movement in fractured rock is extremely complex because contaminants will move along the line of least resistance, which is in the fracture and often in a direction that cannot be determined by conventional methods for hydrogeologic investigations. Because of the tendency of contaminants to move through the fractures to locations that are difficult to determine and to access, remediation of fractured rock aquifers poses an extreme technical challenge.
Ground Water Flow
The major influences on ground water flow are precipitation, which recharges aquifers, and gravity, which causes ground water to flow and eventually discharge to springs, rivers, and oceans. As an example, Figure 2-1 shows recharge and discharge in unconsolidated sediments in an idealized and simplified cross section. Water moves from a recharge area at high elevation to a discharge area at low elevation. Water may also move vertically through a series of less permeable layers, known as confining beds, that may separate aquifers at various depths. Figure 2-2 illustrates the complexities of ground water flow paths for fractured, consolidated rocks. Flow paths in fractured rock are often difficult to ascertain because the fractures are not uniformly distributed and may not be interconnected.
The fundamental law describing ground water flow is known as Darcy's Law, which can be expressed as follows:

In this equation, the term q is the volumetric flow rate per unit cross-sectional area of aquifer perpendicular to the direction of flow. The term K is the hydraulic conductivity (see Table 2-1), which is a measure of the ease with which water moves through the aquifer material and which decreases with pore size (just as the flow of water through a pipe decreases with pipe diameter). The term dh/dl is the hydraulic gradient (see Table 2-1), which quantifies the pressure and gravity forces that drive flow and which is influenced by aquifer recharge, elevation, and pumping. Darcy's Law states that the rate of ground water flow is determined by the magnitude of the hydraulic gradient and the magnitude of the hydraulic conductivity of the aquifer material.
The Effect of Pumping on Flow
Pumping ground water, as is done with pump-and-treat systems, causes complex perturbations in flow, even at sites with relatively simple hydrogeology. To illustrate this effect, consider a hypothetical site at which water is injected into the aquifer at one well and pumped out of the aquifer at the same rate from a second well. Placement of the wells is critical in determining the flow of water from the recharge well to the discharge well. In the top diagram in Figure 2-3, the wells are placed on a line perpendicular to the direction of ground water flow, and only a small amount of the recharged water (indicated by the stippled area) is removed by the pumping well. In the bottom diagram, the wells are aligned parallel to the direction of ground water flow, and nearly all of the recharged water is pumped out, with almost none moving downgradient. At an actual field site, ground water flow is far more complex than in this simple illustration because the hydraulic properties that describe an aquifer are not uniform. Nevertheless, this example demonstrates the necessity of understanding the flow system before installing a well to remove contaminants or to control the direction of movement of a contaminant plume.
Geochemical Characteristics
Once contaminants enter the subsurface, they become subject to control not only by the aquifer's physical properties but also by a variety of possible geochemical reactions. These reactions may cause the contaminants to change form, sorb to aquifer solids, or form complexes with other chemical species. In addition, geochemical characteristics of the site can influence the operation of aquifer cleanup systems. For example,
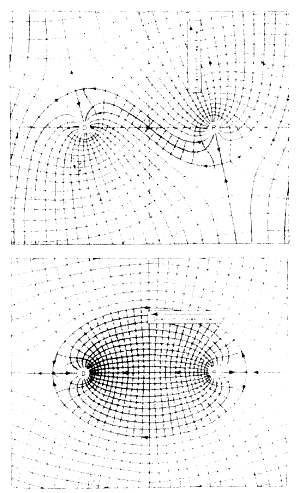
FIGURE 2-3 Diagrams, called flow nets, that illustrate the effect of well placement on the direction and quantity of ground water flow in a homogeneous aquifer with recharge (R) and discharge (D) wells that are at the same depth and are pumping the same amount of water. The solid lines are flow lines, which indicate the pattern of ground water movement. A flow net, by definition, has an equal quantity of water flowing between each pair of flow lines. The dashed lines are lines of equal hydraulic head. Only the water in the stippled areas moves from the recharge well to the discharge well. In the top diagram, the wells are placed in a line perpendicular to the direction of ground water flow, and little recharge water reaches the discharge well. In the bottom diagram, the line from the recharge well to the discharge well is parallel to the direction of ground water flow, and nearly all of the water from the recharge well reaches the discharge well. SOURCE: Da Costa and Bennett, 1960.
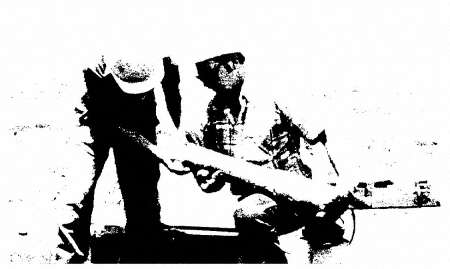
Core barrel used to obtain a sample from the subsurface. Courtesy of Rice University, Department of Environmental Science and Engineering.
geochemical characteristics determine whether metals, such as iron, will precipitate when the contaminated water is extracted for treatment. Metal precipitates can clog extraction wells and require installation of special treatment systems, significantly increasing cleanup costs.
The chemical composition of ground water reflects both the mineralogy of the aquifer and the flow path of the water from the point of recharge to the point of discharge. The movement of water and its interaction with soil and rock can be described by the concept of a hydrogeochemical cycle, shown in Figure 2-4. As the figure indicates, the chemistry of the infiltrating water is largely controlled by temperature, precipitation, soil mineralogy, and anthropogenic inputs, including airborne contaminants and chemicals in runoff water. Factors that affect the chemical composition of the water once it infiltrates the subsurface include the mineralogy of the aquifer matrix, the residence time that the water is in contact with the soil and rock, and mixing of ground water with other sources of water or subsurface contaminants. Contaminants and products from microbial activity in the subsurface can also cause important changes in the water chemistry. As shown in Figure 2-4, as water moves through the unsaturated zone the concentrations of solutes increase as soil gases and minerals (which may contain metals such as iron and manganese) dissolve. Ground water has a longer residence time
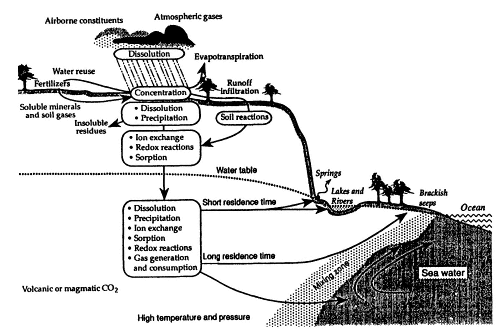
FIGURE 2-4 Schematic showing the hydrogeochemical cycle of water moving through the subsurface. Water begins its path as rainfall, which may contain dissolved matter such as pollutants. Some of this rainfall infiltrates the soil, where the composition of the water changes as soil gases dissolve and as the water reacts chemically with soil components (through ion exchange, redox, sorption, and dissolution reactions). From the soil, the water migrates downward to the water table. Once in the aquifer, the water flows toward discharge points such as springs, lakes, rivers, and the ocean, continuing to change chemically along the way. It then evaporates from these surface bodies and once again begins its path as rainfall. SOURCE: Modified from Back et al., 1993.
in the deep subsurface than in shallow systems and continues to change in chemical composition as it moves to discharge points in springs, lakes, rivers, estuaries, and oceans. In general, shallow aquifers (2 to 50 meters below land surface) are more vulnerable to ground water quality problems than deep aquifers because most contaminants enter the subsurface via use and disposal near the land surface or leakage from buried storage containers.
Table 2-2 summarizes the many types of geochemical processes that can affect contaminant fate and transport in the subsurface. Many of these reactions occur together or sequentially in contaminated environments (Cherry et al., 1984). For example, the oxidation of organic contaminants to carbon dioxide can be coupled with the reduction of (insoluble) ferric iron oxides to aqueous (soluble) ferrous iron. When sulfide is present, the ferrous iron can precipitate as monosulfide or pyrite. These processes occur within the constraints of thermodynamics and in many cases are mediated by microorganisms.
Biological Characteristics
Microorganisms of many kinds, primarily bacteria but also protozoa and fungi, inhabit subsurface environments (Ghiorse and Wilson, 1988). Microorganisms are important in ground water systems because they consume organic matter, including contaminants, and because they alter the chemical state of the aquifer. Subsurface microorganisms are also extremely important in the development of new technologies that use biological processes to treat contaminated ground water in place rather than having to extract it (see Chapter 4).
The activity of microorganisms in uncontaminated aquifers is often limited by the availability of metabolizable organic carbon, which the organisms require for growth and reproduction. The concentration of natural organic carbon is low in ground water (usually less than 2 mg/liter). Therefore, the presence of degradable organic contaminants in the subsurface generally stimulates microbial growth, although some organic compounds and trace metals inhibit microbial activity.
Two broad classes of bacteria play important roles in the subsurface and in the development of new ground water cleanup technologies: aerobic and anaerobic. Aerobic organisms require oxygen to degrade organic compounds. They transfer electrons from the organic material to oxygen, which is termed the ''electron acceptor.'' The organic material is oxidized and the oxygen is reduced. This process generates energy for the organisms and transforms the organic material to carbon dioxide and new cell mass.
Anaerobic organisms use substances other than oxygen as electron
TABLE 2-2 Geochemical Processes in Subsurface Environments
Process |
Definition |
Significance in Aquifers |
Effect on Contaminants |
Dissolution-precipitation |
Reactions that dissolve or precipitate solids such as natural minerals |
Primary control on the chemical composition of ground water |
Can increase or decrease the concentrations of dissolved constituents, including some types of contaminants |
Oxidation-reduction |
Reactions that add electrons to (reduce) or remove electrons from(oxidize) chemicals, altering their chemical form |
Determines the speciation of metals with more than one oxidation state and the possible chemical and biological degradation pathways of organic matter |
Can alter contaminant concentration either by direct chemical reactions or by increasing microbial degradation of the contaminant; can dissolve or precipitate metals |
Sorption-desorption |
Reactions that transfer a substance from the fluid phase (solvent) to the solid phase (sorbent), or vice versa |
Affects dissolved concentrations by the attachment and release of constituents on surfaces of aquifer sediment |
Sorption can slow the movement of contaminants |
Ion exchange |
Exchange of ions in clays for ions in solution, with charge balance maintained |
Reduces the concentration of one ion and increases the concentration of another |
Can remove contaminant ions from solution, particularly when clay is present, and thus slow their removal by pumping |
Complexation |
Interactions between chemicals in solution that generate combined chemical species, such as ion pairs, complex ions, or chelates |
Affects the availability of substances in ground water to participate in reactions |
Can alter the concentrations, reactivities, and mobilities of contaminants (especially metals) |
acceptors when degrading organic compounds. For example, denitrifying bacteria, which are one common class of anaerobes, use nitrate as an electron acceptor, converting the nitrate to nitrite, nitrous oxide, nitric oxide, or nitrogen gas. Similarly, sulfate-reducing bacteria, a second type of anaerobe, use sulfate as an electron acceptor, converting it to hydrogen sulfide. A third class of anaerobes, the iron reducers, transforms ferric iron (Fe(III)) to ferrous iron (Fe(II)). A fourth class of anaerobes, the methanogens, uses simple carbon-containing compounds such as carbon dioxide or acetate as electron acceptors, producing methane. Each of these classes of organisms can oxidize organic materials to simpler compounds like carbon dioxide, in the process gaining energy for growth and reproduction.
Because electron acceptors are as essential for microbial growth as oxygen is for human growth, the availability of electron acceptors is a major factor that influences the extent of microbial transformation occurring in aquifers and the design of treatment technologies relying on biological processes. Uncontaminated ground water in shallow aquifers is nearly saturated with dissolved oxygen. However, when the oxygen supply is depleted due to microbial consumption of organic matter, it is replenished slowly—by oxygen dissolved in water that recharges the aquifer and to a lesser extent by diffusion from the unsaturated zone. Consequently, alternate electron acceptors play an important role in the subsurface. The most common subsurface electron acceptors other than oxygen are nitrate, sulfate, ferric iron, and inorganic carbon. Electron transfer to oxygen generates more energy for the bacteria than electron transfer to these other electron acceptors. Therefore, theoretically, aerobic organisms will dominate in environments where oxygen is present.
Where a sufficient electron acceptor supply and a large source of metabolizable organic carbon are present, the availability of nutrients such as nitrogen, phosphorus, and to a lesser extent sulfur can limit bacterial growth. The bacteria require these and other elemental nutrients to synthesize new cell matter.
CONTAMINANTS IN GROUND WATER
Once a contaminant enters the subsurface, its fate depends not only on the natural physical, chemical, and biological characteristics at the site but also on the chemical properties of the contaminant. Surveys reveal that a wide range of hazardous substances may be present at hazardous waste sites (NRC, 1991). The most common of these substances are in the following classes: volatile organic compounds (VOCs), toxic inorganic compounds, polycyclic aromatic hydrocarbons (PAHs), polychlorinated biphenyls (PCBs), pesticides, and phthalates. Subsurface migration path-
ways, mobility, and persistence of contaminants differ greatly between classes and within each class, primarily due to the different chemical natures of the compounds but also due to their modes of release.
Inorganic Compounds
The most frequently reported hazardous substances include 9 inorganic compounds in the top 25 contaminants (see Table 1-1). These inorganics, in order of their frequency of detection in ground water at hazardous waste sites, are lead (Pb), chromium (Cr), zinc (Zn), arsenic (As), cadmium (Cd), manganese (Mn), copper (Cu), barium (Ba), and nickel (Ni). Of this group, Pb, As, and Cd are also ranked among the top ten most hazardous contaminants.
Anthropogenic sources of inorganic compounds include ore mining and smelting, burning of fossil fuels, petrochemical processing, electronic component manufacturing, and industrial plating. Wastes from these processes that were deposited on land or in ponds, wells, or landfills may release inorganic contaminants to the subsurface. In addition, leaking storage tanks that held leaded gasoline may be sources of lead in ground water.
Detection of inorganic compounds at low concentrations at hazardous waste sites can also result from natural weathering processes or sampling procedures rather than from industrial wastes. With the exception of cadmium, metals may occur naturally in aquifer materials and may dissolve by natural weathering processes. In addition, if during sampling the ground water contacts well screens, casings, or pumps constructed of metal, some metal may dissolve in the water. Another source of metals reported in the parts-per-billion range in ground water is suspended sediment that is analyzed in unfiltered water.
The hazard posed by inorganic contaminants depends on the form in which they are present. For example, the toxicity of Cr and As depends on the oxidation state. Cr(VI) is very toxic, whereas Cr(III) is much less so. Similarly, As(III) is more toxic than As(V). Inorganics that form organometallic ions or compounds have increased mobility and therefore present a greater hazard. For example, Pb, As, and Cr can form organometallic ions or compounds. Table 2-3 lists the oxidation states and principal dissolved forms of four hazardous inorganics.
Organic Compounds
Table 2-4 lists representative organic compounds that are prevalent ground water contaminants and representative properties important to remediation efforts. Such organic chemical contaminants typically enter
TABLE 2-3 Characteristics of Toxic Inorganics in Ground Water
Metal |
Oxidation State(s) |
Principal Dissolved Formsa |
|
Lead |
Pb(II), Pb(IV) |
Pb2+ ion Hydroxide complexesb Carbonate or sulfate ion pairsc Organic (e.g., tetraethyl lead) |
|
Arsenic |
As(III), As(V) |
Arsenate (As5+) oxyanions Arsenite (As3+) oxyanions Organic (e.g., dimethyl arsenic acid) |
|
Cadmium |
Cd(II) |
Cd2+ ion Carbonate ion pairs Chloride, hydroxide complexes |
|
Chromium |
Cr(III), Cr(VI) |
Cr3+ ion Hydroxide complexes (Cr3+) Chromate, dichromate (Cr6+) oxyanions |
|
a From Hem, 1985. b Complex ions are species composed of two or more single ions that are combined (e.g., Pb(OH)3-). c Ion pairs are ions of opposite charge that are adjacent in solution, temporarily forming a pair (e.g., PbCO3(aq)). They are weakly bonded relative to complexes. |
the subsurface as constituents of a liquid phase. This liquid phase may be a dilute aqueous solution, a concentrated leachate, or an organic liquid that is not miscible with water (commonly referred to as a nonaqueous-phase liquid, or NAPL). In addition, the liquid frequently contains mixtures of compounds from various classes, complicating the contaminants' behavior (Department of Energy, 1992).
The characteristics of the "carrier" fluid can greatly influence the migration pathways and persistence of a specific compound. NAPLs, in particular, have been the focus of substantial research and regulatory concern because NAPLs are extremely common and have complicated ground water cleanup at many hazardous waste sites. Contamination by NAPLs occurs in many ways: surface spills; waste injection into subsurface disposal wells; leaking waste disposal lagoons; and leaking drums, pipelines, and storage tanks. As constituents of NAPLs, substantial quantifies of compounds that are only slightly water soluble may travel large distances in the subsurface. VOCs, PCBs, and PAHs usually enter the subsurface as components of NAPLs. Metals also are common constituents of NAPLs; for example, crude oil and solvent wastes from metal plating operations contain metals.
The two most prevalent classes of compounds likely to exist as NAPLs are the chlorinated solvents (such as trichloroethylene and tetra-
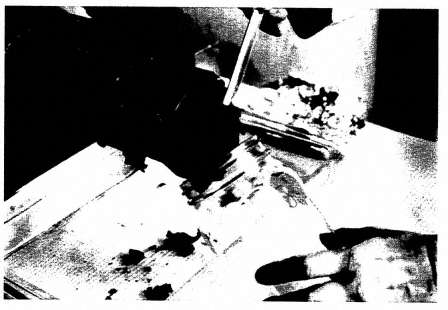
Collection of a sample from a core of material removed from the subsurface. Courtesy of Rice University, Department of Environmental Science and Engineering.
chloroethylene) and petroleum hydrocarbons (including gasoline and fuel oils) (Mercer and Cohen, 1990). Chlorinated solvents are more dense than water and are thus called dense NAPLs, or DNAPLs. Other DNAPLs include coal tars, which contain PAHs, and transformer oil, which may include mixtures of PCBs. Petroleum hydrocarbons are less dense than water and are thus called light NAPLs, or LNAPLs. The mechanics of migration for contaminants vary greatly depending on whether the contaminant is dissolved in water or is carried in an LNAPL or DNAPL.
Compounds having melting points in excess of ambient temperature (approximately 10 to 20°C) may be present as a solid phase and hence immobile in pure organic form; the PAHs listed in Table 2-4 illustrate this behavior. However, such organic solids are likely to dissolve in the liquid organic solvents comprising the NAPL phase, if present.
Mechanics of Migration
Figure 2-5 shows a simplified contamination scenario in which a DNAPL, such as a chlorinated solvent, has been released from a surface
TABLE 2-4 Properties of Representative Organic Ground Water Contaminants
Pollutant |
Aqueous Solubility (mg liter-1) |
Henry's Law Constant (atm m3 tool-1) |
Kow |
Specific Gravity |
Absolute Viscosity (cP) |
Melting Point (°C) |
Chiorinated hydrocarbons |
||||||
Carbon tetrachloride |
7.57 × 102 |
2.41 × 10-2 |
4.37 × 102 |
1.58 |
0.965 |
-23 |
Trichloroethylene |
1.10 × 103 |
9.10 × 10-3 |
2.40 × 102 |
1.47 |
0.566 |
-73 |
Tetrachloroethylene |
1.50 × 102 |
2.59 × 10-2 |
3.98 × 102 |
1.63 |
0.89 |
-23 |
1,2 Dichlorobenzene |
1.0 × 102 |
1.93 × 10-3 |
3.98 × 103 |
1.30 |
1.32 |
-17 |
Fuel hydrocarbons |
||||||
Benzene |
1.75 × 103 |
5.59 × 10-3 |
1.32 × 102 |
0.873 |
0.603 |
5.5 |
Toluene |
5.35 × 102 |
6.37 × 10-3 |
5.37 × 102 |
0.862 |
0.552 |
-9.5 |
p-Xylene |
1.98 × 102 |
7.05 × 10-3 |
1.41 × 103 |
0.861 |
0.644 |
13 |
Oxygenated compounds |
||||||
Bis-2-ethylhexyl phthalate |
2.85 × 10-1 |
3.61 × 10-7 |
9.50 × 103 |
1.21 |
2.14 |
-50 |
Phenol |
9.3 × 104 |
4.54 × 10-7 |
3.0 × 101 |
1.0576 |
1.24 |
43 |
Methyl ethyl ketone |
2.56 × 105 |
4.66 × 10-5 |
2.9 × 10-1 |
0.805 |
0.40 |
-86 |
PAHs |
||||||
Benzo[a]pyrene |
3.8 × 10-3 |
2.4 × 10-6 |
1.00 × 106 |
1.35 |
NA |
177 |
Pyrene |
1.30 × 10-1 |
5.10 × 10-6 |
8.00 × 104 |
1.27 |
NA |
150 |
Naphthalene |
3.17 × 10-1 |
4.60 × 10-4 |
1.95 × 103 |
1.16 |
NA |
80 |
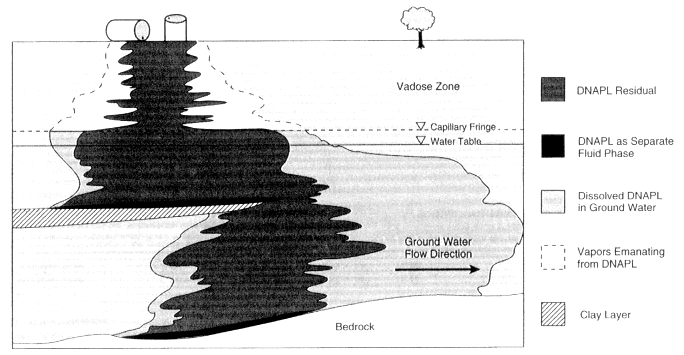
FIGURE 2-5 Conceptual diagram of DNAPL migration through the subsurface. As the diagram indicates, some DNAPL remains as an entrapped residual in the soils (indicated by the dark shading), some migrates as a separate fluid phase (indicated by the black areas), some dissolves in the ground water to create plumes (indicated by the light shading), and some vaporizes into the gas in the soil pores (indicated by white areas).
source. As the chemical enters the subsurface, the liquid may dissolve into the water in the pores, volatilize into the air in the pores, or remain behind in the pore spaces as an entrapped residual. In the unsaturated zone near the ground surface, the organic liquid will move mainly vertically downward with little lateral spreading because gravity forces predominate. The portion that volatilizes, however, will be free to move laterally as an organic vapor within the unsaturated zone, effectively increasing the area of contamination. When the DNAPL reaches the saturated zone (where water completely fills the pore spaces), its downward progress may slow somewhat due to the resistance created by the water. If the spill is sufficient in size, however, it will continue to move vertically downward, displacing the ground water.
In the saturated zone, some of the chemicals from the DNAPL will dissolve in the flowing ground water, forming a contaminant "plume," as illustrated in Figure 2-5. The remainder of the DNAPL will continue to migrate downward, as in the vadose zone leaving behind a trail of residual liquid entrapped in pores. The main body of the DNAPL will continue to move downward until reaching a relatively impermeable stratum, such as the clay layer shown in Figure 2-5. Upon contacting such a layer, the DNAPL may spread laterally, creating a liquid pool. If the impermeable layer is of limited lateral extent, further vertical migration is possible once the DNAPL pool on top of the obstruction extends to the edge. Overall, gravity forces will cause the DNAPL to move predominantly downward, effectively uncoupling its transport from the predominantly horizontal movement of the ground water.
Figure 2-6 shows a second contamination scenario in which an LNAPL, such as a petroleum hydrocarbon, has been released from an underground storage tank. The LNAPL's behavior in the unsaturated zone will be similar to that described above for the DNAPL. When the LNAPL encounters the saturated zone, however, it will spread laterally, forming a "pancake" or "lens" floating at the top of the water table. After its formation, this lens will migrate primarily in the direction of natural ground water flow. If the water table changes due to seasonal fluctuations or pumping, the lens can also spread vertically within the formation, enhancing the area of LNAPL contamination.
The above scenarios illustrate the three most important potential contaminant migration pathways: (1) aqueous-phase transport within the contaminant plume, (2) vapor-phase transport, and (3) transport as a NAPL. The relative importance of each pathway for the transport of a particular contaminant depends on the properties of that substance, as well as the chemical and physical characteristics of the site.
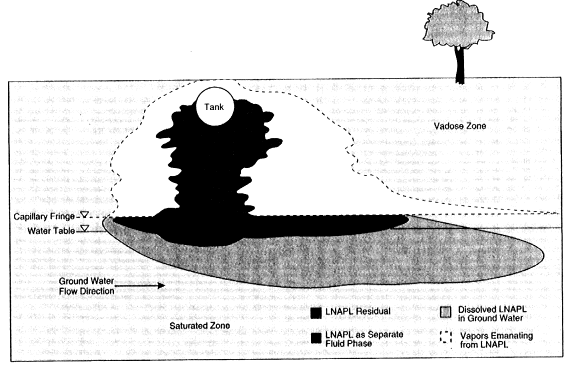
FIGURE 2-6 Conceptual diagram of LNAPL transport through the subsurface. As the diagram indicates, some LNAPL remains entrapped in the soil pores, some remains as a separate fluid phase near the spill source and on top of the water table, some vaporizes into the soil pores, and some dissolves in the ground water to form a plume. SOURCE: Modified from API, 1989.
Aqueous-Phase Transport
The mechanics of aqueous-phase contaminant transport differ for the unsaturated and saturated zones. In the unsaturated zone, flows are gravity driven and thus are primarily vertical. In the saturated zone, flows are primarily horizontal. Saturated-zone transport presents perhaps the greatest opportunity for a pollutant to migrate large distances. Natural ground water flow rates, however, are slow in most formations (1 to thousands of meters per year), so this migration will take place over large time scales—months to hundreds or thousands of years, compared to days or weeks in most rivers and streams.
The propensity for a particular contaminant to be transported in the aqueous phase depends primarily on its aqueous solubility. Solubilities of common organic contaminants vary widely (see Table 2-4) and are influenced by hydrophobicity, temperature, salinity, dissolved organic matter, or the presence of multiple organic compounds in solution (cosolvents). The transport behavior of metals in the subsurface is extremely complex. These substances can occur in many forms—as free metals, ions, complexes, ion pairs, or organometallic compounds each of which has distinct properties. The solubility of metals depends primarily on the pH, the presence of complexing ligands, the redox potential of the solution, and the tendency of the metals to precipitate. For example, Pb(II) reaches its highest concentrations when the ground water is acidic and the bicarbonate concentration is low, such that lead carbonate does not precipitate.
Once a compound dissolves in ground water, it Will migrate in the direction of ground water flow. This migration is called ''advective flux.'' As the contaminant moves through a formation, small- and large-scale variations in the flow field caused by the presence of micro- and macroscopic heterogeneities will tend to spread the contaminant. This process, termed "hydrodynamic dispersion," is illustrated in Figure 2-7. In Figure 2-7a, the soil grains (pore-scale heterogeneities) cause the contaminant to spread as it threads its way through the soil pores. In Figure 2-7b, the effect of this spreading is shown at a larger scale, where the point source is observed to spread in the directions parallel and perpendicular to the average ground water flow direction. Larger-scale heterogeneities (for example, zones of differing permeability) also contribute to, and indeed usually dominate, contaminant spreading.
Although advective and dispersive fluxes play the primary role in the migration of a dissolved contaminant, solutes also migrate from regions of high concentration to regions of low concentration by a process called "molecular diffusion." Diffusion is an extremely slow process in
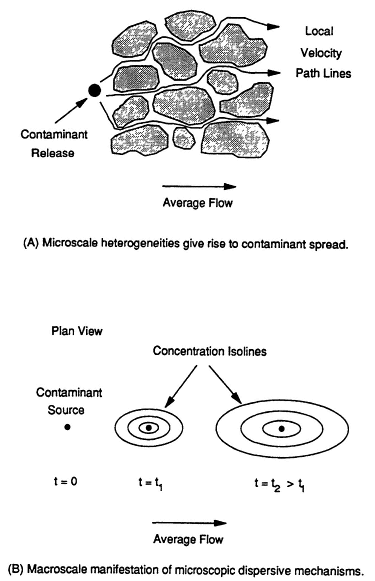
FIGURE 2-7 Conceptual diagrams showing how hydrodynamic dispersion can increase contaminant spreading. (A) shows how the water (and associated contaminants) disperses from the average flow direction as it encounters grains of soil. (B) shows the effect of this spreading on the contaminant concentration at a single location over time (indicated by t). At times t1 and t2, dispersion has caused the contamination to spread in directions perpendicular and parallel to the average direction of ground water flow.
water, but over large time scales it provides a mechanism for the transfer of contaminants to less permeable zones in the aquifer.
While diffusion and dispersion enhance contaminant spreading, they also lower the concentrations of contaminants. For sources of very small volume, such processes, coupled with natural recharge mechanisms, could potentially reduce contaminant concentrations to acceptable levels at some distance from the source. Unfortunately, because acceptable concentration levels are so small (on the order of parts per billion for many contaminants), the required travel distances for sufficient dilution are generally extremely large.
If a compound has a high aqueous solubility, its presence may change the ground water's density and viscosity. Small density increases—as little as 0.1 percent—may cause the contaminant plume to "sink" (i.e., travel deeper into the aquifer) over large travel distances (Sudicky et al., 1983; Mackay et al., 1986). Large density variations can create gravity flow instabilities. Transport behavior in such systems is a subject of ongoing research (Oostrom et al., 1992).
Vapor-Phase Transport
Contaminants that volatilize into the air contained in soil pores can spread laterally and vertically through the unsaturated zone. Subsequent dissolution into flowing ground water or infiltrating recharge water may then enlarge the zone of ground water contamination (Sleep and Sykes, 1992). A contaminant's tendency to volatilize can be determined from the Henry's Law constant, representative values of which are given in Table 2-4. The Henry's Law constant, which is valid for sparingly soluble nonelectrolytes, relates the aqueous-phase concentration of a chemical to its partial pressure in the gas phase. Contaminants with larger Henry's Law constants have a greater tendency to volatilize.
Volatilized contaminants may migrate within the soil gas via advection or molecular diffusion. Advection may occur due to variations in gas-phase density created by the presence of contaminant vapors. Since gases offer small resistances to flow (low viscosities), transport by advection in the soil gas phase is much more rapid than advective transport in ground water. Similarly, transport by diffusion tends to be much more rapid in the soil gas phase than in the ground water. Free air diffusivities, which describe the relative rates at which contaminants migrate by diffusion in air, are several orders of magnitude larger than free liquid diffusivities: 10-2 to 10-1 cm2/s for air, versus 10-6 to 10-5 cm2/s for dilute aqueous solutions.
As soil moisture increases, the importance of vapor-phase transport diminishes. An increase in the moisture content reduces the pore space
available for migration, decreasing the effective diffusivity and gas-phase permeability. In addition, the partitioning of the organic vapor into this increased aqueous-phase volume further retards vapor-phase migration.
NAPL Transport
Bulk NAPL migration is governed primarily by three fluid properties: density, viscosity, and interfacial tension.
Density determines the contaminant's behavior when the spill encounters the saturated zone. LNAPLs tend to spread laterally at the top of the water table, whereas DNAPLs tend to sink vertically. Table 2-4 shows representative NAPL specific gravities.
Viscosity influences the NAPL's rate of migration. Fluids with lower viscosity migrate more rapidly due to their reduced resistance to flow.
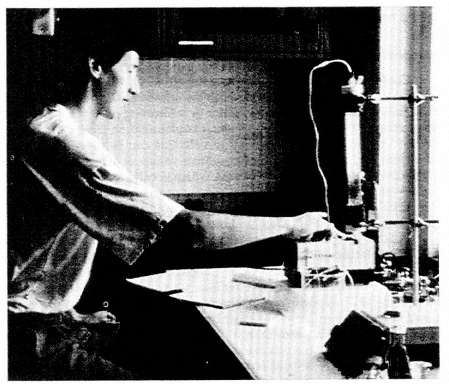
Laboratory experiment to characterize the hydraulics of a column filled with porous material. Courtesy of the Johns Hopkins University, Department of Geography and Environmental Engineering.
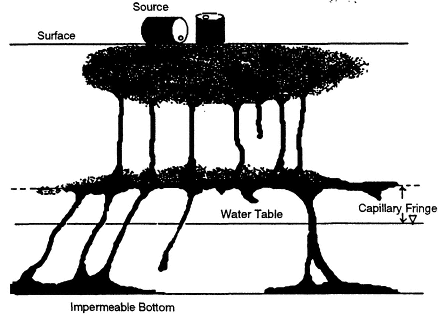
FIGURE 2-8 Enhanced DNAPL migration via viscous fingering. SOURCE: After Schwille, 1988.
Table 2-4 shows that many NAPLs, including some chlorinated solvents and aromatic hydrocarbons, have viscosities smaller than that of water (which is 1.0 centipoise at 20°C). When a denser, less viscous fluid displaces another of lower density and higher viscosity, the process is inherently unstable. Under such circumstances, small perturbations at the displacement front can propagate, creating narrow, preferential pathways of migration for the denser fluid, as shown in Figure 2-8. These pathways are known as "fingers." Fingering can enhance the rate of DNAPL migration. It also makes a spill's location quite difficult to detect because of the small lateral dimensions of the finger.
Interfacial tension gives rise to the capillary forces that control the extent of lateral spreading of a NAPL as it migrates downward. Interfacial tension between two fluids is a measure of the difference in the inter-molecular forces at the interface and the intermolecular forces of the bulk fluids (Adamson, 1982). NAPL-water interfacial tensions are on the order of 20 to 50 dynes/cm (Mercer and Cohen, 1990). The extent and thickness of an LNAPL "pancake" that forms at the water table (see Figure 2-6) decrease and increase, respectively, as the interfacial tension in-
creases. The existence of interfacial forces can also create a barrier to the movement of NAPLs into fine-pore materials; thus, a downward-migrating DNAPL tends to spread out horizontally when it encounters a low-permeability layer, in a manner similar to that shown at the capillary fringe in Figure 2-6. Interfacial tensions can be affected by pH and dissolved constituents such as natural humic substances that behave as surface-active agents, reducing capillary forces.
Overall, the pathways of NAPL migration are intricate, and the resulting contaminant distribution is highly nonuniform and complex. These complexities greatly complicate the task of locating and removing the contamination.
Mechanisms for Retention
Once contaminants are released to the subsurface, a variety of mechanisms act to retain the contaminants beneath the ground. These mechanisms have two effects: they retard contaminant movement, and they create long-term sources for contaminant elution. The propensity of a specific compound to be retained depends on many factors, including its mode of transport (aqueous, gaseous, NAPL), its chemical behavior, and the chemical and physical properties of the porous medium. Two primary modes of retention are sorption and NAPL entrapment.
Sorption and Ion Exchange
The tendency of a contaminant to sorb to solid materials in the subsurface is governed by a number of molecular interactions of chemical, electrostatic, or physical origin. For metals, sorption is generally caused by the electrostatic affinity of the contaminant for an electrically charged surface, such as that of clay, in the porous matrix. Sorbed metals can remain in aquifers for long time periods and can then be released when the geochemistry of the aquifer changes. Small changes in pH or redox conditions, which may occur during remediation, can have profound effects on the amount of metal that sorbs.
For nonpolar organic contaminants, sorption is a function of the compound's hydrophobicity (its affinity or lack of affinity for dissolving in water). Hydrophobicity may be quantified by the octanol/water partition coefficient (Kow) of a compound, shown in Table 2-4 for representative contaminants. The Kow is the ratio of the concentration of the compound that will dissolve in octanol to its concentration in water when equilibrated between these two phases. In general, the higher the Kow value, the greater the propensity for sorption to organic constituents of
the soil. Sorption processes are more complex for soils of very low organic content and for polar and ionic organic solutes.
To estimate the amount of a contaminant that is sorbed to the aquifer solids, mathematical expressions known as sorption isotherms are typically employed. An isotherm is an experimentally determined relationship between the quantity sorbed per unit volume of solid and the concentration in solution. Its application assumes uniform temperature and equilibrium conditions. If the isotherm expression is linear, this implies that the extent of sorption is directly proportional to the dissolved concentration. Over small concentration ranges, a linear isotherm can usually provide a good representation of laboratory data. Over larger ranges of concentration, however, nonlinear expressions are often required.
When a contaminant is released to the subsurface, sorption retards its movement with respect to the ground water (compare the nonsorbing compound and linear sorption curves in Figure 2-9a). Nonlinear sorption will not only retard migration but will also increase the spread (or apparent dispersion) of the contaminant. If contaminant concentrations are measured at a monitoring well downstream of the contaminant release site, sorption will tend to reduce concentration levels and cause the contaminant to appear later at the well (see Figure 2-9b). Nonlinear sorption will create a long period of "tailing," during which contaminant concentrations will decrease slowly (Weber et al., 1991). Thus, under nonlinear sorption conditions, the contaminant will be retained much longer in the porous medium, and much larger volumes of water will be required to flush the system.
The total quantity of a contaminant in a unit volume of aquifer relative to that dissolved in the ground water is termed the "retardation factor." Retardation factors depend on contaminant properties (such as Kow) and soil properties (such as organic carbon content). They vary over a wide range, from a value of 1 for nonsorbing compounds such as chloride ions to as large as 100 or more for strongly sorbing compounds such as PCBs in an aquifer with a high organic carbon content. A retardation factor of 1 for chloride indicates that all of the chloride ions are dissolved in the ground water, and a retardation factor of 100 for PCBs indicates that only 1 percent of the PCBs is dissolved in ground water and 99 percent is sorbed to the aquifer solids. If the amount of a chemical sorbed to the aquifer solids is at equilibrium with the concentration in the ground water, the retardation factor is the coefficient by which one must divide the average ground water velocity to obtain the velocity of the sorbed chemical.
The degree to which equilibrium sorption is a valid assumption is a question that has important implications for remediation. If sorbed contaminants do not equilibrate with the adjacent pore water, then the dura-
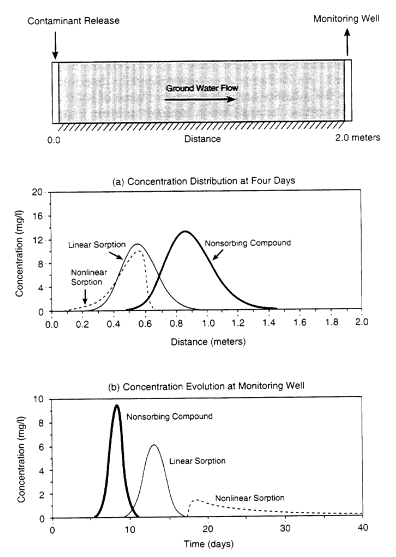
FIGURE 2-9 Influence of sorption on contaminant transport. A contaminant is released at a concentration of 20 mg/liter over a short time period (i.e., in a pulse release); (a) illustrates the concentration profile as a function of distance from the release point four days after release for nonsorbing and sorbing solutes, while (b) illustrates the time evolution of the concentration measured 2 meters downstream of the release point for sorbing and nonsorbing solutes. As shown in (a), sorption slows movement of the contaminant toward the monitoring well. As shown in (b), nonlinear sorption creates a long period of "tailing," during which the contaminant concentration decreases slowly at the well.
tion of cleanup may be extended substantially by the slow desorption. A number of investigations have suggested that sorption processes may exhibit nonequilibrium behavior under natural field conditions (Brusseau and Rao, 1989). Such behavior could be due to the kinetics of the sorption reaction, the mineralogical composition of the formation, or the slow diffusion of contaminants into less accessible pores within the solid materials. Whatever the underlying mechanism, nonequilibrium sorption impedes the release of sorbed contaminants, extending the duration of remediation.
NAPL Entrapment
As a NAPL migrates through the subsurface, small globules become trapped within porous materials by capillary forces. This entrapped NAPL is frequently quantified as the residual saturation (sr): the volumetric ratio of entrapped contaminant to the total pore volume. Entrapment occurs when capillary forces are large enough to overcome the viscous and gravitational or buoyancy forces that would otherwise cause continued contaminant movement. Residual saturation is thus a function of pore geometry, NAPL properties (including interfacial tension, viscosity, and density), ground water flow velocity, and porous medium wettability. Values of sr measured in field-scale and laboratory experiments are typically in the range of 10 to 35 percent in water-saturated, unconsolidated media, with levels as high as 50 percent in materials of low permeability (Conrad et al., 1987; Schwille, 1988). In unsaturated media, residual saturation tends to be 50 to 75 percent smaller (Wilson et al., 1990) due to the reduction in capillary forces in this zone. Graded materials with a wide distribution of particle sizes (and, consequently, pore sizes) tend to entrap more contaminants.
Due to the strength of the capillary forces holding it in place, the entrapped NAPL cannot be mobilized by ground water flow under typical operating conditions for pump-and-treat systems (Darcy velocities in the range of 0.01 to 50 meters per day) (Powers et al., 1991). Thus, contaminants present as residual saturation contamination will be physically removed only by dissolution or volatilization to the ground water or gas phases, respectively. Because NAPLs typically have low aqueous solubilities, removal of entrapped NAPL contaminants by pump-and-treat systems will be a very slow process.
Degradation Reactions
Contaminants can be transformed into other compounds, ionic species, or elemental forms by both microbial and chemical processes in the
subsurface. Degradation rates for organic compounds vary by several orders of magnitude for both microbial and abiotic reactions, even for compounds with similar structures. Thus, to predict the behavior of contaminants in aquifers, reaction rates need to be known for each compound of interest under environmental conditions specific to the aquifer. Contaminants usually degrade by sequences of reactions that occur under different environmental conditions. In some cases, these reactions may terminate before producing stable, harmless end products, leaving hazardous intermediate byproducts. The term "degradation" usually refers to complete transformation all the way to stable, harmless end products, whereas the term "transformation" refers to partial degradation.
Microbial Transformations
Microbial transformations of many organic compounds have been documented in numerous laboratory investigations. In aquifers, however, the possibility of these laboratory-tested reactions occurring depends on hydrologic and geochemical conditions and on the availability of electron acceptors, nutrients, metabolizable carbon, and bacteria capable of mediating the transformations. Some compounds degrade only under aerobic or anaerobic conditions, some under either condition, and others not at all. Table 2-5 lists susceptibility to biodegradation by various classes of bacteria (discussed earlier in this chapter) for five organic contaminants frequently found at hazardous waste sites. For most of these compounds, degradation by aerobic bacteria is faster than degradation by the other types of bacteria. However, the subsurface oxygen supply is often limited, and therefore the activity of the other types of bacteria shown in Table 2-5 often determines the extent of degradation of organic compounds in the anoxic part of a contaminant plume. The estimated half-lives for biodegradation of six monoaromatic hydrocarbons at five methanogenic field sites ranged from 0.05 to 3.6 years (Barker and Wilson, 1992).
Microbial populations may need to adapt before they are able to degrade certain man-made contaminants. The adaptation time may be required for microorganisms to multiply to a significant biomass or to mutate so that they can use the organic contaminant. Organic compounds known to be degradable can at first resist degradation, later disappearing in short time periods after the microbial biomass acclimates. Such a case explained the sudden disappearance of 1,2-dichlorobenzene in a sand and gravel aquifer in a field test reported by Roberts et al. (1986). It is important to realize that while organisms can adapt to most organic contaminants, adaptation may require a long time period. In addition, some
TABLE 2-5 Microbial Degradation of Selected Organic Compounds
contaminants, such as mercury, inhibit microbial growth when the concentration is sufficiently high.
Abiotic Transformations
At some contaminated sites, abiotic chemical reactions (i.e., reactions not caused by microbial activity) can have an important influence on the fate of contaminants. Abiotic reactions can either convert the contaminant to another type of hazardous compound or eliminate it. Transformation rates generally proceed faster by microbial than by abiotic processes, but some abiotic reactions can be fast enough to influence the fate of contaminants in aquifers.
One of the most commonly observed ground water contaminants, 1,1-dichloroethene (see Table 1-1), is formed as the result of an abiotic reaction. This chemical is not widely used. Its prevalence at contaminated sites is due to the abiotic transformation of 1,1,1-trichloroethane, a common solvent, by elimination. Studies have indicated that the half-life of 1,1,1-trichloroethane is between about 3 and 19 years (Vogel and McCarty, 1987).
An important abiotic reaction that can detoxify contaminants is substitution of other groups for halogens. For example, in hydrolysis reactions, a hydroxyl group can replace a halogen to form an alcohol and a hydrogen halide. The half lives of hydrolysis reactions for nine halogenated methanes containing bromide or chloride at concentrations typical for fresh-water systems were 0.05 to 7,000 years (at 25°C and a pH of 7); the median rate was 274 years (Mabey and Mill, 1978). A 1,000-fold reduction in contaminant concentration at this median rate would require 3,000 years. Thus, except for a few compounds, hydrolysis of halogenated hydrocarbons is too slow to be significant in remediation programs.
SPATIAL VARIABILITY
The subsurface is inherently heterogeneous. At the microscale, aquifers contain a mixture of multiple phases—solid, liquid, and gas—characterized by complex geometry. At larger scales, natural geologic processes that occurred during the formation of the aquifer and subsequent chemical, physical, and biological processes result in multiple levels of heterogeneity. The impact of such heterogeneities on contaminant migration and aquifer remediation cannot be overemphasized. Heterogeneities result in the transport of contaminants to places where they are difficult to locate and remove, and they cause the accumulation of contaminants in zones that subsequently become long-term sources of pollution.
Physical Heterogeneity
At the microscale, an aquifer may be composed of aggregates of materials that have large pore spaces between the aggregates but small pore spaces within the aggregates. The presence of such aggregates can substantially affect contaminant transport. Dissolved contaminants will tend to migrate via molecular diffusion into the small pores within these aggregates (often referred to as immobile zones). Once present within the aggregates, these compounds will serve as a long-term source of pollution, as they slowly diffuse from the pores when the contaminant concentration in the mobile ground water is reduced (Van Genuchten and Wierenga, 1976; Rao et al., 1980). Such mobile-immobile zone mass transfer processes mimic the behavior described above for sorption. Both processes tend to create a persistent contaminant release mechanism, interfering with attempts to flush contaminants out of the aquifer.
Microscale heterogeneities can also influence NAPL migration and entrapment. Recent studies have revealed that grain size variability can affect the entrapment and subsequent dissolution of organic globules (Powers et al., 1992). Nonuniform media tend to impede the dissolution of entrapped NAPLs more than uniform materials of the same average grain size. As with sorption and diffusion from aggregates, this slowing of the dissolution process further enhances the persistence of the contaminants underground.
Larger-scale heterogeneities also pose complications. For example, in sand and gravel aquifers, deposits of silt and clay are common. Because water moves more slowly through silt and clay than through sand and gravel, silt and clay layers can significantly change ground water and contaminant movement from what would occur in a sand and gravel aquifer without silt and clay deposits. Heterogeneities can cause wide variability in hydraulic properties such as hydraulic conductivity. For example, in a detailed investigation of a so-called ''homogeneous'' sand and gravel aquifer at a scale of approximately 1,000 m3, the hydraulic conductivity varied between 0.001 and 0.021 cm/s (Sudicky, 1986). The geologic features that controlled the variability were at a scale of a few centimeters to tens of centimeters vertically and one to a few meters horizontally. These changes in hydraulic properties enhance the dispersion of a dissolved contaminant as it travels over large distances, increasing the contaminant spread. Due to the presence of large-scale heterogeneities, the spreading of dissolved contaminants at the field scale is generally much greater than would be predicted by employing laboratory-scale dispersion information (Anderson, 1979). Accurate prediction of contaminant transport at a specific site thus requires a detailed (on the scale of 10 to 100 cm) description of aquifer hydraulic properties. As an exam-
ple, Figure 2-10 illustrates the complexity of a bromide plume produced by a tracer test conducted in a relatively uniform sand and gravel aquifer in Cape Cod, Massachusetts. The complexity of the plume, as determined from 650 multilevel samplers, reflects the sensitivity of transport to spatial variations in hydraulic properties even in simple subsurface environments.
The presence of low-permeability zones or lenses can cause rate-limited mass transfer behavior similar to that observed in aggregated soils. Over time, solutes will diffuse into such strata. These zones thus function alternately as sinks and then sources of solute mass for the bulk ground water (Valocchi, 1988; Gilham et al., 1984). The large amounts of time required for compounds to diffuse out of these low-permeability strata can add considerably to the time and volume of water required to flush out contaminants (Wilson, 1992).
The presence of low-permeability lenses will also influence NAPL migration. For materials with sufficiently small pores, NAPL phases cannot displace the water in the pores and therefore cannot penetrate the pores. These strata therefore act as capillary barriers to NAPL flow, inducing lateral spreading, irregular migration pathways, and "pooling" of the NAPL (see Figures 2-5 and 2-6). Such pools may be extremely difficult to locate. In addition, ground water makes minimal contact with these NAPL pools, thus causing flushing to be an ineffective method for recovering the organic liquid.
Heterogeneities can also create preferential pathways for NAPL migration, greatly enhancing travel distance and limiting lateral spreading. NAPLs have been observed to preferentially flow along fine-scale surfaces between subsurface layers (Kueper et al., 1993). In addition, small-scale permeability variations can trigger the onset of viscous fingering in an inherently unstable flow regime. Subsurface macropores, created by plant roots or chemical weathering, and formation joints and fractures can serve as preferential pathways. In addition to enhancing the contaminant transport rate, such pathways may permit the NAPL to penetrate otherwise impermeable strata and facilitate the transport of contaminants between formations. Preferential flow paths tend to create highly variable distributions of NAPL in the subsurface. Under such conditions, locating contaminants is a formidable task, making contaminant removal through excavation infeasible and posing great difficulties for contaminant containment.
Chemical Heterogeneity
Variable chemical composition within a formation may also affect contaminant transport over a range of scales. At the pore scale, a vari-
able mineral composition will create a complex environment in which many sorption mechanisms may coexist (Weber et al., 1992) and behavior is not easily quantified. At a larger scale, spatial variability in organic carbon content or formation mineralogy will tend to produce zones of high contaminant retention. Some theoretical studies suggest that spatial variability of chemical factors controlling sorption can enhance contaminant dispersion (Garabedian, 1987; Valocchi, 1989). Relatively little is known, however, about the degree to which sorption characteristics vary and are correlated with variations in hydraulic conductivity (Sudicky and Huyakorn, 1991). Variable mineral composition and organic carbon content may also affect NAPL entrapment mechanisms, possibly creating zones of entrapped NAPL that are less accessible to flowing ground water.
Source Variability
In addition to physical and chemical variability, variability in the composition and distribution of the contaminant source also affects contaminant behavior in the subsurface. Contaminants rarely enter the subsurface individually. Thus, an understanding of the behavior of mixtures is critical to predicting contaminant migration and retention. Unfortunately, the composition of the contaminant source is usually imprecisely known. For NAPLs, properties such as density, viscosity, solubility, and interfacial tension depend on composition. For example, chlorinated solvents ordinarily enter the subsurface as DNAPLs, which tend to sink below the water table, but they may also enter as minor components of a hydrocarbon mixture constituting an LNAPL, which tends to spread laterally at the water table. As components of a NAPL mixture dissolve or volatilize over time, the NAPL properties change, a process termed "weathering." Very little information is available pertaining to the weathering of chemical mixtures of interest in aquifer remediation.
The presence of multiple dissolved contaminants creates competition for sorption sites. In severely contaminated systems, the properties of dissolved contaminants will also be influenced by the presence of cosolvents. The effect of multiple solutes and cosolvents on sorption phenomena seriously complicates the task of characterizing contaminant distribution and transport (Weber et al., 1991; Nkedi-Kizza et al., 1985; Rao et al., 1990).
The magnitude, temporal evolution, and spatial distribution of the source of contamination greatly influence the transport and retention of pollutants. These factors, however, are unknown at many sites. Temporal fluctuations in contaminant release will create zones of high concentration within a plume, although such zones tend to flatten over large
travel distances due to natural dissipative mechanisms. The size of the spill may influence the amount of contamination that ultimately reaches the aquifer. For example, if a spill is small, it may never reach the saturated zone due to residual entrapment of the contaminant within the unsaturated zone. The spatial distribution of the original source of contamination may affect the extent of aquifer contamination. For example, DNAPLs will tend to remain localized within a small horizontal cross-sectional region because these chemicals have no tendency to spread laterally until they encounter a stratum of low permeability.
In the majority of cases it is useful to separate the contamination conceptually into two parts: (1) the plume of dissolved contaminant and (2) contaminant source areas, which include the potentially substantial amounts of contaminant in precipitated or NAPL form. As Chapter 3 will explain, the plume can be contained and its size decreased by pumping. The sources of contamination are generally much more difficult to control and remove; they will often extend over a considerable volume, and no adequate methods are available for locating all of them. Removal of contaminant sources by pumping may require years or centuries, depending on factors such as the solubility of the contaminant in water, the size of the sources and their distribution, and the flow pattern of the aquifer.
RESEARCH NEEDS FOR IMPROVING UNDERSTANDING OF THE CONTAMINATED SUBSURFACE
This chapter has documented that the subsurface environment even in its uncontaminated condition—is a complex system that scientists do not fully understand. Advances in knowledge about subsurface processes are essential for improving ground water contamination assessments and cleanup technologies. These advances are needed in three broad areas: subsurface characteristics, contaminant transport and distribution, and reaction pathways and rates. The greatest progress will be made if site cleanups are accompanied by investigations aimed at identifying the critical conditions and processes controlling contaminant behavior, while gathering data helpful for optimizing performance of the cleanup system.
Subsurface Characteristics
This chapter has documented that hydrogeologic investigations of contaminated environments must provide both fine resolution and complete coverage of the contaminated area. Heterogeneities ranging from
the microscale to the macroscale can have profound effects on transport of contaminants and performance of cleanup systems. Errors in predicting subsurface characteristics—and hence the performance of cleanup systems—frequently result from the inability to obtain representative samples at both sufficiently small and large scales. Improvements in subsurface sampling methods especially in noninvasive methods that do not jeopardize the integrity of the site with extensive drilling—would significantly advance the ability to design ground water cleanup systems and to predict the fate of contaminants. Research is needed to answer the following questions:
-
How can sampling methods be improved to ensure that the samples are representative?
-
What parameters—physical, chemical, and biological—must be included in the site characterization program for formulation of realistic cleanup objectives and optimization of the treatment system?
-
How can the variability of aquifer properties be characterized over a sufficient range of scales—millimeters to kilometers—to understand the effect of variability on contaminant transport and cleanup system performance?
-
What level of characterization is needed to support decisionmaking at the various stages of remedial investigation and design?
-
Can the variability of aquifer properties be assessed adequately with statistical information developed from common geologic environments?
-
How can the costs of sampling methods be reduced to allow more extensive sampling at sites?
-
What new ideas and techniques can contribute to development of a reliable three-dimensional map of subsurface geology and ground water flow patterns at a site?
Contaminant Distribution and Transport
Considerable progress has been made in recent years in developing the ability to predict the distribution and transport of contaminants in the subsurface. For example, a greater understanding now exists pertaining to the coupling of permeability variations and dispersive contaminant transport. Similarly, the fundamental physical phenomena governing NAPL migration and entrapment have been identified in laboratory studies under simple, well-defined conditions. However, much still needs to be learned regarding contaminant behavior in complex natural systems. Most investigations of contaminant fate in the subsurface have been carried out under ideal conditions such as in homogeneous aquifers
with single contaminants. As this chapter has emphasized, however, the subsurface is neither chemically, biologically, nor physically homogeneous. Unfortunately, relatively little is understood about the impact of heterogeneities on processes that control the fate and transport of contaminants, including sorption, abiotic and biotic reactions, and residual entrapment and dissolution. More information is needed about the behavior of contaminants at a fundamental level in nonideal systems to answer the following questions:
-
How can the full range of contaminants and other organic compounds in the ground water—not just those targeted in the monitoring plan—be determined?
-
What critical parameters and properties govern transport of contaminants in various types of complex subsurface systems?
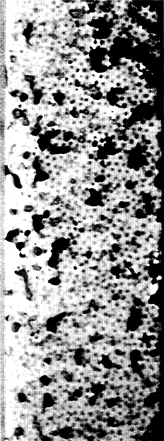
Trapped globules of NAPL in a laboratory column containing a porous medium (glass beads). Courtesy of Rice University, Department of Environmental Science and Engineering.
-
How can the relationships between these critical parameters and properties and the transport of contaminants be quantified?
-
How can these properties be measured, or how can they be inferred from more easily quantifiable media properties?
-
How can detection methods for NAPLs or other concentrated sources of contamination be improved to provide more detailed characterization of the distribution of contaminant mass?
-
How can data from sampling and pumping wells be used to locate contaminant sources more precisely?
-
How can site-specific data best be incorporated into mathematical models for predicting contaminant transport?
Reaction Pathways and Rates
This chapter has documented that a variety of chemical and biological reactions influence the fate of contaminants in the subsurface. Time scales for ground water transport are generally large enough—on the order of many years—that even exceedingly slow reactions can register an impact. For this reason, classes of reactions of minimal importance in other environments can significantly affect the fate of ground water contaminants. Furthermore, the large fluid-solid interfacial area typical of aquifers increases the occurrence of reactions mediated by mineral constituents and microorganisms on the solid surfaces.
The heterogeneity of subsurface conditions greatly complicates understanding of contaminant reactions. Because of the diversity of geochemical conditions, expressed as compositional gradients from the molecular scale to the macroscale, a bewildering variety of reaction behavior can occur. Furthermore, the study of subsurface reaction rates poses severe methodological problems, especially when working with natural aquifer solids of diverse composition. Special precautions are necessary to define and control the experimental system sufficiently so that reaction rates can be measured while at the same time preserving the connection to the real environment. It is not surprising that researchers have been reluctant to take up this challenge and that knowledge is often sparse regarding the reaction mechanisms and pathways that may determine the fate of ground water contaminants. The fundamental study of such reaction systems is relatively new, and if pursued may open new vistas for in situ ground water cleanup. Research is needed to address the following questions:
-
What are the critical chemical and biological reactions affecting contaminants in various types of subsurface environments?
-
How can rates of these reactions be quantified, and how do they
-
depend on geochemical conditions and the presence of particular microorganisms?
-
How can reaction rates measured in the laboratory be extrapolated to the field?
-
Are naturally occurring microorganisms able to achieve desired biotransformations, or must specially cultured organisms be introduced?
-
How can the subsurface microorganisms be assayed to enable prediction of biochemical transformations?
-
To what extent do specific chemical or biochemical reactions depend on geochemical conditions such as pH, redox state, and mineralogy?
-
To what extent do particular reactions modify geochemical conditions—pH, redox state, and surface composition—thus promoting or inhibiting other reactions?
-
For localized contaminant sources, what chemical treatments could destroy or solubilize contaminants, particularly NAPLs?
-
How can reaction rate information best be incorporated into mathematical models for predicting contaminant fate?
CONCLUSIONS
Based on consideration of the properties of the subsurface environment and contaminant behavior in the subsurface, the committee reached the following conclusions:
-
Theoretically, it is possible to clean up contaminated ground water, subject to constraints imposed by the principles of mass and energy conservation, thermodynamics, and kinetics. However, practical limitations arising from the uneven distribution of contaminants, subsurface complexity, and the inherently slow rate of ground water movement severely restrict decontamination efforts.
-
Subsurface environments have complexities and heterogeneities that make them inherently difficult to decontaminate. The complexity of the subsurface and the difficulty of characterizing it contribute in large measure to the problems experienced in ground water cleanup documented in the following chapters. While some generalizations are possible, each site has a character of its own and must be studied carefully to enable effective remediation.
-
Contaminants found at hazardous waste sites are diverse in nature, manifesting a wide range of properties that may complicate remediation. Contaminants that are present as separate liquid phases, sorbed strongly to aquifer soil and rock, or precipitated as solids constitute a large reservoir that is difficult to remove. Immiscible liquid contami-
-
nants (NAPLs) especially increase the complexity of remediation because they migrate into places inaccessible to extraction by hydraulic forces.
-
Ground water contamination problems may become increasingly complex with the passage of time because of the potential for contaminants to migrate and accumulate in less accessible zones. Measures to remove contaminants from zones where the release occurred and to contain contaminants that cannot be removed should be taken as soon as possible after the contamination occurs.
-
Transport and transformations in the subsurface occur relatively slowly, at time scales as long as years, decades, and centuries. All of those concerned with ground water remediation—scientists and technologists, as well as decisionmakers and the public—must recognize that ground water cleanup requires patience and perseverance to an extent considerably greater than for surface water cleanup. Expectations of quick and easy solutions are illusory.
REFERENCES
Adamson, A. W. 1982. Physical Chemistry of Surfaces, 4th ed. New York: John Wiley and Sons.
Anderson, M. 1979. Modeling of ground water flow systems as they relate to the movement of contaminants. CRC Crit. Rev. Environ. Control 9:97-156.
API (American Petroleum Institute). 1989. A Guide to the Assessment and Remediation of Underground Petroleum Releases, 2nd ed. Publication No. 1628. Washington, D.C.: API.
Back, W., M. J. Baedecker, and W. W. Wood. 1993. Scales in chemical hydrogeology: a historical perspective. Pp. 111-129 in Regional Ground Water Quality, W. Alley, ed. New York: Van Nostrand Reinhold.
Barker, J. F., and J. T. Wilson. 1992. Natural biological attenuation of aromatic hydrocarbons under anaerobic conditions. Pp. 57-58 in Proceedings of the Subsurface Restoration Conference, Dallas, Texas, June 21-24 . Houston: Rice University.
Brusseau, M. L., and P. S. C. Rao. 1989. Sorption non-ideality during organic contaminant transport in porous media. CRC Crit. Rev. Environ. Control 19:22-99.
Cherry, J. A., R. W. Gilham, and J. F. Barker. 1984. Contaminants in groundwater: chemical processes. Pp. 46-64 in Groundwater Contamination: Studies in Geophysics. Washington, D.C.:National Academy Press.
Conrad, S. H., E. F. Hagan, and J. L. Wilson. 1987. Why are residual saturations of organic liquids different above and below the water table? In Proceedings—Petroleum Hydrocarbons and Organic Chemicals in Groundwater: Prevention, Detection and Restoration. Worthington, Ohio: National Water Well Association.
Da Costa, J. A., and R. R. Bennett. 1960. The pattern of flow in the vicinity of a recharging and discharging pair of wells in an aquifer having areal parallel flow. Pp. 524-536 in General Assembly of Helsinki: Commission of Subterranean Waters Publication No. 52. Gentbrugge, Belgium: International Association of Scientific Hydrology.
DOE (Department of Energy). 1992. Chemical Contaminants on DOE Lands and Selection of Contaminant Mixtures for Subsurface Science Research. Report No. DOE/ER-0547. Washington, D.C.: DOE.
EPA (Environmental Protection Agency). 1982. Aquatic Fate Process Data for Organic Priority, Pollutants. Report No. 440/4-81-014. Washington, D.C.: EPA.
Garabedian, S. P. 1987. Large-Scale Dispersive Transport in Aquifers: Field Experiments and Reactive Transport Theory. Ph.D. dissertation. Massachusetts Institute of Technology, Department of Civil Engineering, Cambridge, Mass.
Ghiorse, W. C., and J. T. Wilson. 1988. Microbial ecology of the terrestrial subsurface. Adv. Appl. Microbiol. 33:107-172.
Gilham, R. W., E. A. Sudicky, J. A. Cherry, and E. O. Frind. 1984. An advection-dispersion concept for solute transport in heterogeneous unconsolidated geological deposits. Water Resources Res. 20(3):369-372.
Heath, R. C. 1980. Basic Elements of Ground-Water Hydrology with Reference to Conditions in North Carolina. U.S. Geological Survey Water Resources Investigations Open-File Report 80-44. Washington, D.C.: U.S. Government Printing Office.
Heath, R. C. 1983. Basic ground-water hydrology. U.S. Geological Survey Water Supply Paper 2220. Washington, D.C.: U.S. Government Printing Office.
Hem, J. D. 1985. Study and Interpretation of the Chemical Characteristics of Natural Water. U.S. Geological Survey Water Supply Paper 2254. Washington, D.C.: U.S. Government Printing Office.
Kueper, B. H., D. Redman, R. C. Staff, S. Reitsma, and M. Mah. 1993. A field experiment to study the behavior of tetrachloroethylene below the water table: spatial distribution of residual and pooled NAPL. Ground Water 31(5):756-766.
LeBlanc, D. R., S. P. Garabedian, K. M. Hess, L. W. Gelhar, R. D. Quadri, K. G. Stollenwerk, and W. W. Wood. 1991. Large-scale natural gradient tracer test in sand and gravel, Cape Cod, Massachusetts: 1. experimental design and observed tracer movement. Water Resources Res. 27(5):895-910.
LeGrand, H. E. 1988. Region 21, Piedmont and Blue Ridge. Pp. 201-208 in Decade of North American Geology: GNA 0-2, Hydrogeology, W. Back, P. R. Seaber, and J. S. Rosenshein, eds. Boulder, Colo.: Geological Society of America.
Lucius, J. E., G. K. Olhoeft, P. L. Hill, and S. K. Duke. 1992. Properties and Hazards of 108 Selected Substances. Open File Report 92-527. Golden, Colo.: U.S. Geological Survey.
Mabey, W., and T. Mill. 1978. Critical review of hydrolysis of organic compounds in water under environmental conditions. J. Phys. Chem. Ref. Data 7:383-415.
Mackay, D. M., D. L, Freyberg, P. V. Roberts, and J. A. Cherry. 1986. A natural gradient experiment on solute transport in sand aquifers 1: approach and overview of plume movement. Water Resources Res. 22:2017-2029.
Mercer, J. W., and R. M. Cohen. 1990. A review of immiscible fluids in the subsurface: properties, models, characterization and remediation. J. Contain. Hydrol. 6:107-163.
Montgomery, J. H., and L. M. Welkom. 1990. Groundwater Chemicals Desk Reference. Chelsea, Mich.: Lewis Publishers.
Nkedi-Kizza, P., P. S. C. Rao, and A. G. Hornsby. 1985. Influence of organic cosolvents on sorption of hydrophobic organic chemicals by soils. Environ. Sci. Technol. 19:975-979.
NRC (National Research Council). 1991. Environmental Epidemiology, Volume I: Public Health and Hazardous Wastes. Washington, D.C.:National Academy Press.
Oostrom, M., J. S. Hayworth, J. H. Dane, and O. Given. 1992. Behavior of dense aqueous phase leachate plumes in homogeneous porous media. Water Resources Res. 28(8):2123-2134.
Powers, S. E., C. O. Loureiro, L. M. Abriola, and W. J. Weber, Jr. 1991. Theoretical study of the significance of nonequilibrium dissolution of nonaqueous phase liquids in subsurface systems. Water Resources Res. 27(4):463-477.
Powers, S. E., L. M. Abriola, and W. J. Weber, Jr. 1992. An experimental investigation of NAPL dissolution in saturated subsurface systems: steady-state mass transfer rates. Water Resources Res. 28(10):2691-2705.
Rao, P. S. C., R. E. Jessup, D. E. Rolston, J. M. Davidson, and D. P. Kilcrease. 1980. Experimental and mathematical description of nonadsorbed solute transfer by diffusion in spherical aggregates. Soil Sci. Soc. Am. J. 44:684-688.
Rao, P. S. C., L. S. Lee, and R. Pinal. 1990. Cosolvency and sorption of hydrophobic organic chemicals. Environ. Sci. Technol. 24:647-654.
Roberts, P. V., M. N. Goltz, and D. M. Mackay. 1986. A natural gradient experiment on solute transport in a sand aquifer, 3: retardation estimates and mass balances for organic solutes. Water Resources Res. 22:2047-2058.
Schwille, F. 1988. Dense Chlorinated Solvents in Porous and Fractured Media. Translated by J. F. Pankow. Chelsea, Mich.: Lewis Publishers.
Sleep, B. E., and J. F. Sykes. 1992. The influence of infiltrating wetting fronts on transport of volatile organic compounds in variably saturated porous media. In Proceedings of the International Conference on Subsurface Contamination by Immiscible Fluids (International Association of Hydrology), Calgary, Alberta, Canada, April 18-20, 1990, K. U. Weyer, ed. Rotterdam: A. A. Balkema.
Sudicky, E. A. 1986. A natural gradient experiment on solute transport in a sand aquifer: spatial variability of hydraulic conductivity and its role in the dispersion process. Water Resources Res. 22:2069-2082.
Sudicky, E. A., and P. S. Huyakorn. 1991. Contaminant migration in imperfectly known heterogeneous groundwater systems. Contributions in hydrology: U.S. national report 1987-1990. Pp. 240-253 in Proceedings of the Twentieth General Assembly of the International Union of Geodesy and Geophysics, Vienna, Austria, August, 1991.
Sudicky, E. A., J. A. Cheng, and E. O. Frind. 1983. Migration of contaminants in ground water at a landfill—a case study, 4: a natural-gradient dispersion test. J. Hydrol. 63 (1/2):81-108.
Valocchi, A. J. 1988. Theoretical analysis of deviations from local equilibrium during sorbing solute transport through idealized stratified aquifers. J. Contain. Hydrol. 2(3):191-207.
Valocchi, A. J. 1989. Spatial moment analysis of the transport of kinetically adsorbing solutes through stratified aquifers. Water Resources Res. 25(2):273-279.
Van Genuchten, M. Th., and P. J. Wierenga. 1976. Mass transfer studies in sorbing porous media I: analytical solutions. Soil Sci. Soc. Am. J. 40:473-480.
Vogel, T. M., and P. L. McCarty. 1987. Rate of abiotic formation of 1,1-dichloroethylene from 1,1,1-trichloroethane in groundwater. J. Contain. Hydrol. 1(3):299-308.
Weber, W. J., Jr., P. M. McGinley, and L. E. Katz. 1991. Sorption phenomena in subsurface systems: concepts, models and effects on contaminant fate and transport. Water Res. 25(5):499-528.
Weber, W. J., Jr., P. M. McGinley, and L. E. Katz. 1992. A distributed reactivity model for sorption by soils and sediments: conceptual basis and equilibrium assessments. Environ. Sci. Technol. 26(10):1955-1962.
Wing, S. G., and J. W. Weaver. 1991. Dense Nonaqueous Phase Liquids. Ground Water Issue Report No. EPA/540/4-91-002. Washington, D.C.: EPA.
Wilson, J. L., S. H. Conrad, W. R. Mason, W. Peplinski, and E. Hagan. 1990. Laboratory Investigations of Residual Liquid Organics from Spills, Leaks and Disposal of Hazardous Wastes in Ground Water: Final Report. Report No. EPA/600/6-90/004. Washington, D.C.: EPA.
Wilson, J. L. 1992. You don't need DNAPLs for pump-and-treat to fail. Presentation to the National Research Council's Committee on Ground Water Cleanup Alternatives, Las Vegas, Nevada, September 29, 1992.