3
The Demand Side: Hydrogen End-Use Technologies
The transition to a new energy carrier requires a series of investments and enhancements not only in energy supply and distribution, but also in vehicles and other end-use technologies. This chapter addresses the demand side in three major categories, namely, transportation, stationary power, and industrial uses. Transportation demand scenarios are postulated for the present (i.e., 2002), the near term (2020), and the long term (2050).
In the 1970s a number of studies, driven by energy-independence considerations, predicted that a hydrogen economy might emerge as early as the year 2000. Today the interest in a transition to a hydrogen economy is driven not only by concerns about energy security but also by those about global climate change and air quality. Rapid improvements in the proton exchange membrane fuel cell (PEMFC) during the past decade have been a catalyst for this renewed interest in a hydrogen economy because of the fuel cell’s potential in transportation applications. In this chapter, the nature and magnitude of demand for hydrogen (H2) are examined in a number of categories, with special attention focused on customer and regulatory attributes. (See Chapter 5 and Appendix E for estimates of well-to-wheels energy use.) On the basis of these analyses, technology barriers are identified that will need to be addressed in the DOE’s research, development, and demonstration (RD&D) activities.
The focus of most of this report and this chapter is on light-duty passenger vehicles, the largest segment of the vehicle market. Stationary power systems to produce electric power from hydrogen may be an important part of a possible future H2 energy system, both in a transition to a hydrogen economy and also in the steady state. The committee did not do an extensive analysis of the future stationary electric power system in the United States and the role that H2 may play, but the section below entitled “Stationary Power: Utilities and Residential Uses” delineates some of the developments and opportunities in fuel cells and turbines for stationary power.
TRANSPORTATION
Background and Barriers
The transition to new fuels and/or energy carriers is especially problematic in the transportation sector because of the diffuse nature of the system and its complex public-private composition. Considering land vehicles only, there are more than 750 million passenger cars and commercial vehicles worldwide, with an annual production rate of 56 million units in 2001 (Ward’s Communication, 2002). The geographically diffuse distribution of vehicles favors fuels that are easy to transport and store—that is, fuels that are liquid at room temperature. Consider, for instance, that natural gas fuels and electricity are generally less expensive (on a per unit of energy basis) and tend to be “cleaner” than liquid fuels are, but they are much more difficult to transport, in the case of natural gas, and much more difficult to store, in the case of electricity. Alcohol fuels are easy to transport and store, but they tend to be more expensive than are petroleum fuels, natural gas, and electricity. Most vehicular fuels continue to be gasoline and diesel fuels. The convenience of the petroleum-based fuel distribution system is a key factor in the continuing dominance of vehicles running on liquid fossil fuels. It explains in large part why gasoline hybrid electric vehicles1 (GHEVs) have been successful in penetrating the consumer market, while grid-connected electric vehicles (including grid-connected hybrid electric vehicles) have not.
Because of the large number of vehicles on the road and their relatively slow turnover, a change in fuel and/or energy carrier must be transitional—that is, sufficient fuel must be available for the large existing fleet while the new fuel is introduced in parallel. The success of energy-efficient GHEVs is instructive in two ways: (1) it demonstrates the huge challenge in moving beyond the relatively simple gasoline system now in widespread use, and (2) it creates an even greater barrier to newer technologies, such as the hydrogen fuel cell vehicle (FCV), by enhancing the fuel economy of “conventional” vehicles. The major “demand parameters” for a light-duty vehicle are shown in Table 3-1.
Transportation applications of fuel cell technology and hydrogen fuels not discussed in this report include urban buses, heavy-duty truck auxiliary power units (APUs) (Lutsey et al., 2003; Winter and Kelly, 2003), delivery vehicles, forklifts, airport baggage-handling vehicles, mining vehicles, golf carts, scooters, boats, and even airplanes. Of these, the hydrogen-fueled urban bus market segment has received the most attention.
Fuel Cell Vehicle Technology
The success of hydrogen in the transportation sector will be dependent on the development and commercialization of competitive FCVs. The challenge is to develop automotive fuel cell systems that are lightweight and compact (i.e., have high power densities by both mass and volume), tolerant to rapid cycling and on-road vibration, reliable for 4000 to 5000 hours or so of noncontinuous use in cold and hot weather, and able to respond rapidly to transient demands for power (perhaps by being hybridized with a battery or ultracapacitor for electrical storage on the vehicle), and able to use hydrogen of varying purity.
TABLE 3-1 Key “Demand Parameters” for a Light-Duty Vehicle
Demand Category |
Parameter |
Customer |
Initial cost Operational and maintenance costs Quality Range (between refueling) and refueling convenience Passenger/cargo space Performance (acceleration, speed, ride quality, acceptably low levels of noise, vibration, and harshness) Safety |
Regulatory |
Emissions of pollutants (carbon monoxide [CO], oxides of nitrogen [NOx], hydrocarbons [HC], particulates) Fuel efficiency Greenhouse gas emissions Safety |
One of the most important attributes for FCVs is fuel efficiency, since less fuel means lower fuel costs, less expensive and bulky on-board hydrogen storage, and less upstream environmental impact. Wang (2002) summarizes the numerous studies comparing the fuel efficiency and life-cycle impacts of FCVs, hybrid electric vehicles (including GHEVs), and potential “transition vehicles” with baseline gasoline and diesel vehicles. Ignoring life-cycle impacts, fuel cells operating on hydrogen are much more energy-efficient than are internal combustion engine (ICE) systems. It is impossible to specify accurately how much more efficient they are, since fuel cells have very different efficiency characteristics (e.g., they are many times more efficient at low speeds and loads, but are less efficient at higher speeds and loads) and because automotive fuel cell systems are in their technological infancy and so their future performance cannot be accurately predicted.
For the purposes of quantitative comparisons, after extensive deliberation and literature review, the committee selected a fuel-efficiency improvement factor of 2.40 for FCVs versus a baseline gasoline vehicle—that is, today’s gasoline vehicles are assumed to use two-and-a-half times as much energy as a comparable FCV. This comparison, an average for all light-duty vehicles, is based on average U.S. driving conditions. (For detailed assumptions, see Wang [2002].) The committee selected a fuel-efficiency factor of 1.45 for GHEVs versus a baseline gasoline vehicle. (See the discussion of hybrid technology in the following subsection, “Market Acceptance and Demand Trajectories.”) Fuel-efficiency factors for diesel-powered hybrid electric vehicles would fall between 1.45 and 2.40. These assumptions of fuel economy are based on averages from Wang’s (2002) review of other studies. In practice, actual differences in fuel economy may vary considerably. For instance, automakers might take advantage of the on-board electricity capability of FCVs and introduce a range of high-energy-consuming appliances and services, which would dramatically increase fuel consumption. Alternatively, FCVs might have relatively higher fuel economy because they disproportionately replace gasoline vehicles in urban settings or because traffic congestion results in slower driving speeds—in both cases taking advantage of FCVs’ better fuel efficiency at lower speeds.
Given these requirements, hybrid and nonhybrid PEMFC systems are the leading contenders for automotive fuel cell power, with additional attention focusing on the direct-methanol fuel cell (DMFC) version of the technology and the possibility of using solid oxide fuel cell (SOFC) systems as auxiliary power units for cars and trucks.
An important attraction of all of these fuel cell systems, both as main vehicle power systems and as APUs, is their ability to support the new wave of vehicle electronics that is being introduced. New or planned electronic gadgetry on vehicles includes navigation systems; extensive on-board communications; voice-actuated controls; exterior alternating current (ac) power supplies; computer-controlled, power-
assisted active suspension; collision-avoidance systems; electric air-conditioning compressors; “drive-by-wire” steering; side and rear-view bumper cameras; electronic tire pressure control; and generally greater computer power for increasing control of the various vehicle systems. The need for these systems has already started a trend toward a new 42-volt (V) standard for vehicle auxiliaries in order to deliver more power. In principle, electric (fuel cell) vehicles and APUs provide an efficient way to meet these power demands.
Fuel cell vehicles are attractive potential replacements for ICE vehicles because they can offer performance similar to that of conventional vehicles, along with several additional advantages. These advantages include better environmental performance; quiet (but not silent) operation; rapid acceleration from a standstill, owing to the torque characteristics of electric motors; and potentially low maintenance requirements. Furthermore, FCVs have the potential to perform functions for which conventional vehicles are poorly suited, such as providing remote electrical power (for construction sites, recreational uses, and so on) and possibly even acting as distributed electricity generators when parked at homes and offices and connected to a supplemental fuel supply. FCVs also provide additional attractions to automakers: by eliminating most mechanical and hydraulic subsystems, they provide greater design flexibility and the potential for using fewer vehicle platforms and therefore more efficient manufacturing approaches.
Market Acceptance and Demand Trajectories
For the FCV to be successful in the marketplace, it must satisfy customer desires and regulatory requirements (see Table 3-1). Fuel cell vehicles will easily meet a few of these desires and requirements. They will excel in fuel economy and emissions reduction. On the negative side, for the fore-seeable future they will likely be expensive, have less range, and be more difficult to refuel. Their ability to satisfy other demands and requirements is more ambiguous, depending on perceptions, design decisions, and near-term engineering improvements.
For early fuel cell systems to succeed in the marketplace, they must have special appeal in some market niches, even if these niches are relatively small. One niche might be created by the desire, especially in dense urban areas, to achieve zero tailpipe emissions. The only zero-emission vehicle type other than the direct-hydrogen FCV that is practical at the present time is the battery electric vehicle (EV), which is characterized by short driving ranges, long recharge times, and high costs. To the extent that zero-emission vehicles are encouraged or even mandated in certain areas, direct-hydrogen FCVs may have to compete only with battery EVs and not the entire suite of vehicle technology options. Such a situation could give them a much firmer foothold for breaking in to motor vehicle markets. Another niche might be made up of individuals and businesses that value the large amounts of electrical power carried on board, and that might find a suite of new uses that can only be imagined at this time. And still other niches could include those wanting APUs on trucks or off-road vehicles in areas where noise or pollution is a concern.
One important feature of FCVs that remains crucial for their development is the fact that PEM fuel cells run on either pure hydrogen or a dilute hydrogen gas “reformate” stream (though direct-methanol fuel cells, still in an early stage of development, operate on methanol). This hydrogen can either be stored on board the vehicle in one of several ways, or generated from another fuel with an on-board reformer.
To aid the transition to FCVs without major infrastructure changes, the energy and automotive companies have been working together to develop on-board reformers. On-board reformers convert a liquid (or other gaseous fuel) to hydrogen. Natural gas reforming is more difficult than liquid reforming, and thus the focus has been on liquids for on-board reformers. The most effort has been devoted to methanol and gasoline. DaimlerChrysler was a leader in developing an on-board methanol reformer, and the company unveiled prototype FCVs operating on methanol in the late 1990s. Other companies focused on gasoline reforming. But by 2003, all major automakers had suspended their development of on-board reformers and shifted their FCV efforts to direct hydrogen use. Several oil companies are known to be continuing their development of on-board reformers, which is an appropriate technology to be developed in an industrial R&D laboratory.
On-board reformers are attractive in that they obviate the need to build a hydrogen infrastructure. Methanol is easier to reform than gasoline is, but DaimlerChrysler and others suspended methanol reforming in part because of the challenge of developing a large-scale infrastructure for what was viewed as an interim fuel. More generally, gasoline (and methanol) reforming efforts were suspended by automakers because of several major disadvantages: on-board reformers impose substantial additional cost, add considerable complexity, reduce fuel efficiency, increase emissions, increase “engine” start-up times, and create additional safety concerns. Automakers and others considered these disadvantages to be too large to overcome the advantages of ready gasoline availability, especially when on-board reforming is considered an interim strategy until hydrogen is broadly available.
Most analysts agree that storing hydrogen on board FCVs is the best ultimate solution, but no hydrogen storage system has yet been developed that is simultaneously lightweight, compact, inexpensive, and safe. Further advances in hydrogen storage, so that FCVs can refuel quickly and have driving ranges comparable with those of conventional vehicles, thus constitute a key area for further development. Prototype FCVs have been built that store hydrogen as a cryogenic
liquid, as a compressed gas, in metal hydrides, and as sodium borohydrate. (See Chapter 4 for further discussion of hydrogen storage options.)
Market-related aspects of diesels and consumer electronics also deserve mention. Major sales of GHEVs to date have been in Japan and the United States, because Europe has embraced diesel engine technology in recent years as its major fuel-efficiency solution; in the near term (next decade), this trend will likely continue. However, it should be mentioned that European auto manufacturers were also the first to develop major hydrogen programs—for example, BMW with hydrogen ICEs and DaimlerChrysler with fuel cells and hydrogen ICEs—and these programs are continuing.
In many cases, marketplace competition in rapidly changing technologies speeds up the pace of development. A recent example is the development of batteries for consumer electronics applications. During the past decade, most major battery improvements have been driven by the high-volume need for portable power for laptops and cellular phones, among other devices. Some predict that a similar improvement scenario may occur with fuel cells, because today’s rechargeable batteries cost approximately $3000 per kilowatt (kW) and have much less energy density than do fuel cells. In fact, a recent article in the New York Times noted that methanol-powered fuel cells for laptops might be available within a year (Feder, 2003).
Assuming continuing progress in fuel cell development and the availability of fuel, what are possible scenarios for FCV sales? The recent introduction of GHEVs provides insight. Indeed, the commercialization trajectory of GHEVs provides a “best case” penetration scenario (assuming no major surprises). That is, GHEVs provide a best case because the vehicle attributes were similar to those of a standard, high-volume gasoline ICE vehicle; no fueling infrastructure changes were required; the component technologies were relatively mature; the vehicles were viewed as high-tech and environmentally friendly; and tax benefits aided initial price reductions for the consumers. Table 3-2 shows the actual sales of hybrids through December 2002.
TABLE 3-2 Hybrid Electric Vehicle Sales in North America and Worldwide, 1997 to 2002
Sales Volume |
Year |
|||||
1997 |
1998 |
1999 |
2000 |
2001 |
2002 |
|
North Americaa |
0 |
0 |
0 |
9,600 |
20,700 |
35,900 |
Worldwide |
300 |
17,700 |
15,500 |
24,200 |
42,100 |
59,300 |
Total to dateb |
300 |
18,000 |
33,500 |
57,700 |
99,800 |
159,100 |
aNorth American sales—almost all in United States. bTotal to date: cumulative worldwide sales. SOURCE: Personal communication of committee member Daniel Sperling with Toyota and Honda, 2003. |
Winter and Kelly (2003) address future possibilities. For example, Toyota has a goal of selling 300,000 GHEVs by 2005, and General Motors Corporation (GM) indicates that it will make hybrid technology “available” on a wide variety of models by 2007. Under the most optimistic scenarios for GHEVs, after a decade in production, the annual volume might approach 2 million vehicles, with a total of 4 million GHEVs on the road. In practice, GHEV sales forecasts are being reduced as of this writing. Toyota and Honda continue to expand sales, but GM and Ford have delayed introduction of their initial offerings. A fundamental concern is cost. Toyota declared in 2003 that it was making a profit on its GHEV, the Prius, selling it at about $3500 more than the cost of a comparable conventional gasoline-fueled vehicle. It is expected that this cost premium will gradually drop over time as sales volume increases and learning takes place. Indeed, the 2004 Prius is more powerful and bigger, with better fuel economy and lower emissions, and sells for the same price as the previous-generation Prius.
The cost premiums for GHEVs are in part a function of the technology used in hybrid vehicles. The Prius is known as a “full” hybrid, in the sense that it relies on a large battery pack and large motor for much of its power and energy. Other models to be introduced by Toyota and other automakers will have smaller batteries and electric motors, implying lower cost and also smaller fuel economy improvements relative to conventional gasoline-fueled ICE vehicles. Full hybrids provide up to a 50 percent fuel economy improvement, while “mild” hybrids, with perhaps only an integrated starter/alternator, will provide only about a 10 percent improvement.
In Chapter 6, it is assumed that GHEVs will represent 1 percent of U.S. sales by 2005 and will increase 1 percentage point per year for the next 10 years and 5 percentage points per year for the following 10 years. The energy efficiency that is used for all hybrids is 45 percent improvement relative to conventional gasoline-fueled ICE vehicles.
The committee estimates that the fuel cell system, including on-board storage of hydrogen, would have to decrease to no more than about $100/kW before a scenario even close to the hybrid scenario postulated here would be realized. The most optimistic estimates project 2010 as the year in which $100/kW can be achieved2 (Arthur D. Little, 2001) (although this committee has not had the opportunity to evaluate the basis of such estimates—for example, by conducting a part-by-part cost analysis). As the DOE manages its hydrogen program, it is imperative that it understand the components of these cost estimates and, on the basis of these understandings, appropriately evolve its RD&D programs. Because industry is actively pursuing RD&D in fuel cells, particular
DOE attention should be devoted to related fundamental and exploratory research at universities and national laboratories.
Assuming an optimistic scenario for FCVs and numbers of vehicles entering the marketplace similar to those of GHEVs, FCVs could reach 1 percent of U.S. sales by 2015, and then increase by 1 percentage point per year until 2024 and by 5 percentage points per year thereafter until they dominate the market. (It should be noted that the DOE multi-year program plan for hydrogen RD&D [DOE, 2003b] designates 2015 as the year for a “commercialization decision.”) Figure 3-1 shows the detailed projection for this scenario. The projection takes into account reasonable transitions for the buildup of GHEV and FCV manufacturing and the associated phaseout of conventional and GHEV manufacturing (see Chapter 6).
Thus, by 2020, the total number of FCVs on the road would be fewer than or equal to 4 million units if the optimistic GHEV penetration scenario was matched. Four million vehicles could not justify a national fuel infrastructure change, although regional infrastructure needs might be high as a result of clustered demand growth; that is, in most locations, marketplace demand would not be the main element in a fuel change by 2020.
The committee’s market trajectory for hydrogen fuel cell vehicles reflects what is possible and shows initial market penetration in 2015, growing to 12 percent of new light-duty vehicles sold in 2020 and 40 percent in 2030. Although not directly comparable, there are several other studies that can be compared with the committee’s vision of what might happen. For instance, Argonne National Laboratory (Santini et al., 2003) made a market penetration analysis of FCVs that shows 1 percent market share in 2011, growing to 26 percent in 2020, 52 percent in 2025, and reaching 100 percent in 2038. A report of the Pew Center on Global Climate Change (Mintzer et al., 2003) posits similarly high initial market penetration, but slower increases over time—reaching 2.5 percent penetration in 2015 and 5 percent in 2020, and steadily inching upward to 20 percent annual sales in 2035. The 2003 DOE program (DOE, 2003b) assumes initial penetration in 2018, increasing to 27 percent in 2020 and to 78 percent in 2030.
If the committee’s FCV projection above is “close to actuality” (or even shifted by some number of years into the future), it indicates that a great deal of thought must be given to the fuel service station scenarios for the decade when FCVs grow from a few thousand to a few hundred thousand—that is, in 2010 to 2020, as shown in Figure 3-1. Today the United States has a dense network of about 180,000 retail fuel stations, serving more than 200 million vehicles. Dense coverage, similar to the number of diesel fuel stations in the United States today, will be required as FCVs grow into the millions. Other parts of this report address technolo-
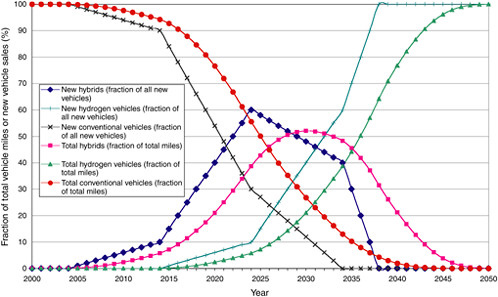
FIGURE 3-1 Possible optimistic market scenario showing assumed fraction of hydrogen fuel cell and hybrid vehicles in the United States, 2000 to 2050. Sales of fuel cell light-duty vehicles and their replacement of other vehicles are shown.
gies that could be employed to bring the relatively large number of retail hydrogen fuel stations online before an extensive network of hydrogen pipelines may be required to be developed.
STATIONARY POWER: UTILITIES AND RESIDENTIAL USES
Introduction
As indicated above, this report and the committee’s analysis have been focused on hydrogen production technologies and the demand for hydrogen in the light-duty-vehicle sector. The use of hydrogen in stationary applications may also play an important role in a hydrogen economy, but it was not analyzed in detail by the committee. Distributed generation (DG) of electrical power is projected by some analysts to be a substantial market. Fuel cells and turbines using hydrogen could provide an important future opportunity for hydrogen produced from sources other than natural gas in areas where pipelines are available. This opportunity could help to stimulate hydrogen infrastructure investments, possibly before FCVs reach commercial readiness.
The U.S. electric power system is projected to use large amounts of coal and natural gas for the next 20 years and to produce a significant portion of the nation’s CO2 emissions (EIA, 2003). Advanced fossil-fueled energy plants of the future could produce electricity and/or hydrogen, and achieve high efficiencies using advanced turbines and fuel cells, while also sequestering by-product CO2 (NRC, 2003b). Hydrogen could be exported from such large plants and used in industrial facilities, either to generate electricity onsite or for process heat; thus, the industrial sector could represent another demand, further stimulating a market for hydrogen production. The committee did not analyze these options and various trade-offs for the use of hydrogen in stationary applications, but the technologies that might be used in stationary applications are addressed in the remainder of this chapter.
In 2001, the U.S. electric power system included about 10,400 generating stations of greater than 60 megawatts (MW), with a total installed capacity of 786 gigawatts (GW). Electricity generation contributes about 40 percent of the CO2 emissions in the United States (see Figure 2-7 in Chapter 2)—largely as a result of coal’s being the source for 50 percent of electricity generation; natural gas is the source for 17 percent. Of the new electricity generation capacity being installed, 80 percent is projected to be with natural gas (EIA, 2003). In comparison with building a comparable fraction of new coal-fueled power plants, this change would reduce carbon emissions, but increase energy demand and imports for natural gas.
Distributed generation is modular generation. DG units are less than 60 MW in size and usually located near the point of use. Technologies available for DG include industrial and aero-derivative gas turbines, reciprocating engines, microturbines, wind turbines, biomass-based generators, solar power and photovoltaic systems, and fuel cells. These technologies offer a greater degree of fuel flexibility than large central power stations do. There are an estimated 10.7 million DG units in place in the United States, of which over 99 percent are small emergency/standby reciprocating engines that are not interconnected with the grid. Currently, 85 percent of the DG units are reciprocating engines; they are fueled primarily by distillate fuel oil or gasoline; combustion turbines make up 5 percent and are fueled by natural gas; and steam turbines constitute 9 percent (Resource Dynamics Corporation, 2003).
DG can be either grid-connected or operated independent of the grid. The aggregate capacity of all DG units in the United States is 169 GW, which is 17 percent of U.S. electricity generation capacity (Resource Dynamics Corporation, 2003). A total of 70 GW of capacity installed prior to 1990 was still operating by the end of 2000. DG units can be used to meet baseload power requirements and needs for peaking power, backup power, remote power, power quality, and heating and cooling. They typically must be able to operate between 40,000 and 50,000 hours without major system overhauls. The market for DG is typically the commercial sector, including hospitals, supermarkets, restaurants, universities, and shopping malls; manufacturing facilities, which need reliable energy; or remote locations where grid power is not available. DG can be customer- or utility-owned.
Direct use of H2 in stationary systems would provide a new fuel option for DG. It could provide a route for a transition to H2 that was produced economically but during the time when FCVs were not ready for commercial introduction.3 The use of hydrogen reformed from natural gas is not likely to displace direct use of natural gas in stationary systems. It is more energy-efficient to use natural gas directly than to convert it to hydrogen in stationary DG applications. (Natural gas is currently the preferred fuel for new DG.)
If economic, small electrolyzers coupled with distributed power-generating devices could replace and supplement batteries in the DG backup power market. So-called regenerative systems in the 1.50 kW scale could convert and store grid power in the form of H2 that could then be used to regenerate power in fuel cells or in combustion devices. This application may represent a higher-value niche for electrolysis and fuel cells than the transportation market does. It might potentially offer less demanding technical challenges: vehicular fuel cells will be subject to vibration and thermal stresses, whereas stationary backup applications would not, and also would need only short-term reliability.
Fuel cells are currently being developed for distributed generation; most are for applications under 1 MW. Some solid oxide fuel cell/gas turbine hybrid systems are being developed for 5 MW applications. Aside from fuel cells used
in space applications, stationary fuel cells (phosphoric acid) are the only fuel cells for which there is “production” and market experience. Stationary requirements are usually less stringent than those of transportation with respect to price ($500/kW versus $50/kW) and footprint, but require longer life (40,000 to 50,000 hours versus 3,600 hours).
Central Power
Direct Fired
Gas turbine engines with a conventional natural gas combustion system or water injection combustion system can operate on H2 or H2-rich fuels with little or no modifications to the core injectors. Modifications to the fuel delivery system and injectors are required. The volumetric heating value of hydrogen is 10,787 kJ/N-m3 (274 Btu per standard cubic foot [scf]) as compared with 35,786 kJ/N-m3 (909 Btu/scf) for methane. In order to supply the required energy input rate to the gas turbine, approximately 3.32 times the volume of hydrogen fuel has to be injected into the primary zone of the combustor to provide the same heating value as that of natural gas fuel.
Large turbines, particularly integrated gasification combined cycle (IGCC) systems, have been run successfully on syngas with volumes up to 62 percent H2 in process plants in the United States and Europe. For example, General Electric (GE) has 10 IGCC projects running on H2-rich fuel, with 6 more planned or going into operation. Nine of these projects are associated with refinery operation (Jones and Shilling, 2002; Jon Ebacher, General Electric Power Systems, “SOFCs, Direct Firing, Wind,” presentation to the committee, April 23, 2003).
Marketplace Scenarios
Since H2 can be burned in gas turbines, these turbines could provide an early market for additional H2 production—assuming that the H2 is not generated from natural gas. Turbines located at the site of the hydrogen production could generate electricity, which could be transmitted via the usual electrical transmission and distribution (T&D) system to residential, commercial, and industrial users.
For this market there are R&D needs to address issues that include the following: (1) combustion technology to reduce NOx emissions and achieve higher efficiencies, (2) fuel management and controls for operability and safety requirements, (3) cost-and-efficiency trade-offs, (4) material compatibility of components with H2 combustion gas, and (5) systems development and optimization.
Fuel Cells for Distributed Generation
Fuel cells offer the potential for very efficient, clean, and quiet distributed power generation. Because the power generation process in fuel cell systems is electrochemical, no emissions from combustion are produced from the power generation itself. These benefits have led to significant federal R&D funding over the past 25 years. Nevertheless, fuel cells are currently more than four times more expensive to install than ICE generators and more than twice as expensive to install as microturbine generators, with which they are frequently compared. The high capital costs of fuel cell systems that have been sold or demonstrated to date have been a major barrier for penetration into the DG market. There are four different fuel cell systems, characterized by their electrolytes, that are potentially suitable for stationary power (Lipman and Sperling, 2003; Shipley and Elliot, 2003). Table 3-3 provides current performance parameters for the various fuel cell types; Table 3-4 presents parameters projected for 2020.
The Energy Information Administration (EIA, 2003) estimates that electricity generation will increase by 2 percent per year to meet increased electrical demands. In 2020, 1.5 trillion kWh of additional electricity-generation capacity will be needed. If 10 percent of the added generation (150 billion kWh) were from hydrogen, it would require 10 million tons of hydrogen, and 20 million tons per year of CO2 emissions might be avoided, assuming that H2 is produced from sources other than coal or natural gas or, if other fossil fuels are used, that the CO2 is sequestered (DOE, 2003a). Of course, existing DG technologies such as microturbines will continue to improve both economically and in terms of achieving higher efficiency; thus, competing technologies are a continual moving target.
The major technical and cost issues for fuel cells regardless of electrolyte or temperature range are (l) stack cost and life, (2) reformer (where needed), and (3) power electronics and overall system integration. Addressing these issues requires basic electrochemistry and material studies. Total funding by the Office of Fossil Energy for its fuel cell activities (phosphoric acid fuel cell [PAFC], MCFC, SOFC) from FY 1978 through FY 2000 was $1167 million (NRC, 2001a), which was cost-shared 20 to 50 percent with industry. The NRC (2001a) study concluded that these funds still did not result in fuel cells’ being commercial.
The Office of Energy Efficiency and Renewable Energy has supported PEM stationary fuel cell R&D since FY 2000 and has spent $22 million. The SOFC and MCFC programs are supported by the Office of Fossil Energy and are not part of the DOE hydrogen program, but are considered “associated programs,” since they are being developed to operate on natural gas and synfuels. However, these programs could be modified and fueled with H2 if it were available.
The following subsections treat various types of fuel cells, currently market-deployed or under development, and discuss them in the context of distributed generation, while noting other applications.
TABLE 3-3 Stationary Fuel Cell Systems—Typical Performance Parameters (Current)
TABLE 3-4 Stationary Fuel Cell Systems—Projected Typical Performance Parameters (2020)
Phosphoric Acid Fuel Cells
The 200 kW phosphoric acid fuel cell (PAFC) was introduced into the market in 1991 by International Fuel Cells/ ONSI, now called UTC Fuel Cells. It is the only commercialized fuel cell technology. PAFC units have been installed in various applications—commercial, small industrial, land-fill, and military—and some are used for cooling, heating, and power. To date there have been 250 units sold, at roughly $4500/kW. The U.S. Department of Defense (DOD) has cost-shared the purchase of three-quarters of the units sold to date. The units have performed well: they have operated at 95 to 98 percent availability and 99.99 to 99.9999 percent reliability and have served 4 million customers and accumulated 4 million hours of operation. The cost of PAFC units has not decreased and in fact has increased from $3500/ kW. These units are not cost-competitive with other DG options, which can provide the same reliability and high-quality power efficiency. Recently, UTC Fuel Cells decided not to manufacture more units and to sell only those in inventory. Current units will continue to be serviced.4
What are the lessons learned from the failure of PAFC to become a commercial success and how do these lessons apply to other stationary fuel cell systems in development and demonstration? Was the cause of failure only the high cost relative to the other DG systems? The PAFC systems appeared to perform well. The federal government had spent more than $411 million on PAFC. Should it have continued
to sponsor R&D to reduce the cost of the commercialized systems? It is often thought that the government can be an early adopter of technology to enable initial volumes to be manufactured and sold. For PAFC, DOD subsidized three-quarters of those produced. Should the government (e.g., the General Services Administration or DOD) make larger purchases of new technologies? Even if costs had been reduced, there are market and regulatory barriers that apply not only to fuel cells, but also to other new DG technologies, such as microturbines.
Proton Exchange Membrane Fuel Cells
The proton exchange membrane fuel cell, which is the fuel cell being considered for vehicle transportation applications, can also be used in DG applications, particularly for small-scale residential and commercial purposes. The PEMFC operating temperature of 150°F is lower than that of the PAFC and much lower than the operating temperatures of the other fuel cell systems in development: the solid oxide fuel cell and the molten carbonate fuel cell. This means that the PEMFC could be used for residential hot water, but not for high-quality steam or combined heat and power (CHP) applications. Many companies (Plug Power, Avista, Ballard, H Power) have been exploring the use of the PEMFC for the 1 to 25 kW market—which would involve residential buildings, including some small multifamily homes. The PEMFC is also being considered in the 50 to 250 kW range. Ballard’s first commercial fuel cell product, the 1.2 kW Nexa® power module, was introduced in the market in 2001. Ballard has introduced the Air Gen Unit at 1.2 kW for backup and intermittent-duty applications; this unit has both hydrogen cylinders and cartridges to supply the hydrogen. Ballard’s first continuous stationary fuel cell will be introduced in Japan in limited volume by the end of 2004 as a 1 kW CHP unit. PEMFC applications can be considered as a niche market, particularly in the under-25-kW size, because in this size range the PEMFC must compete with existing DG technologies that have heating and cooling system applications and are reliable, durable, and low-cost. If there were a sizable market, DG could provide PEMFC manufacturing experience, enhancing the learning curve for PEMFC and hastening its automotive application, which has much more stringent volume and cost requirements. DG applications require longer life than automotive applications do.
The DOE issued a solicitation in January 2003 for the development of stationary PEMFC for buildings, with the target cost of $1500/kW, design life of 40,000 hours with less than 10 percent degradation, and market entry within the next 3 to 5 years.5 Recently UTC Fuel Cells announced that it will introduce 150 kW PEMFC units at $1500/kW in early 2004.6 The company is currently beta testing these units. The Electric Power Research Institute (EPRI) and UTC are currently cofunding a 750 kW PEMFC demonstration that will consist of five 150 kW modules, each with its own processing system, for a projected installed cost of $2600/kW and expected efficiency of 31 percent. The intent is to gain manufacturing experience that would be applicable for PEM automotive fuel cell systems to meet the $50/kW automotive cost target in the 2010 to 2020 time frame. By 2010, UTC expects to have developed an SOFC system, which would be more attractive for DG applications. PEMFCs for stationary applications have similar R&D needs to those for automotive applications, with additional technical challenges related to higher durability (at 40,000 to 50,000 hours), heat utilization (a higher-temperature membrane is needed), power electronics, rapid start-up time for backup power, fuel processing, and development of non-precious-metal catalysts and thermal and water management technologies.
Solid Oxide Fuel Cells
Solid oxide fuel cells have an electrolyte that is solid ceramic and can operate at up to 1000°C. Unlike PEMFC and PAFC systems, there are no noble metals in the anode or cathode. SOFCs can be configured in a tubular or planar configuration and can be operated at high enough temperatures to eliminate a fuel reformer. SOFCs reject high-value waste heat useful for a steam bottoming cycle or available for CHP. These fuel cells can operate on a variety of fuels, including H2, but current SOFCs are being designed for natural gas as the fuel. There is potentially a broad spectrum of power-generation applications, from small, lightweight, compact devices in the range of watts to kilowatts to larger SOFC/turbine hybrid systems in the megawatt range.
In 2001, the DOE Office of Fossil Energy and industry jointly initiated a Solid State Energy Conversion Alliance (SECA) Program for further SOFC development; the program currently involves six industrial teams. In addition, a parallel core technology program is under way at national laboratories and universities. This effort is to be a $500 million, 10-year program to produce modular, mass-produced fuel cells for stationary, transportation (APUs), and military markets. By 2010, the goal is for the SOFC to have 40 to 50 percent efficiencies and to cost less than $400/kW. The SOFC stack represents 30 percent of projected costs; fuel and air handling are another 30 percent.7
In addition to the SOFC as a stand-alone DG or in a CHP system, SOFCs are being developed in an SOFC/gas turbine
hybrid configuration.8 In the hybrid configuration, the fuel cell converts fuel—for example, either direct hydrogen, syngas from fossil fuels, or biofuels—into electricity and water along with by-product heat. The residual fuel from the fuel cell is then burned by a gas turbine for additional electricity production. The product could be in the 1 to 10 MW range with greater than 65 percent efficiency and with fuel flexibility, and it would be cost-effective when compared with the cost of today’s technology.
Applications of the SOFC could include larger commercial sites, industrial manufacturing facilities, and utility substations. As part of its Vision 21 Program, the DOE is sponsoring several hybrid programs, including a 5 MW system (4 MW SOFC, 1 MW gas turbine). GE expects this product to enter the market in 2013. The SOFC hybrid program will utilize the technology advancements from the SECA Program, but there are specific R&D needs related to the hybrid regarding performance, reliability of the life of the stack under a system pressurized operating environment; and optimized system design, controls, and components.
With respect to the marketplace, SOFC and SOFC/gas turbine hybrids are potentially an attractive basis for an efficient, clean, cost-competitive DG system, but they do not depend on having H2 fuel. However, they could facilitate a transition to a H2 economy by making use of H2 for distributed electricity and CHP, while other fuel cells for vehicles are becoming cost-effective, reliable, and efficient. It is important for the DOE to monitor the milestones and goals of the SECA Program and to fully fund it.
Molten Carbonate Fuel Cells
Molten carbonate fuel cells use a mixture of carbonates that are liquid at operating temperature—600°C to 650°C. MCFC, like SOFC, operates at a higher temperature than the PEMFC does; it does not require a fuel reformer; and it can be operated with a hydrogen-rich fuel. The MCFC’s liquid electrolyte means more handling issues. It does not have the ability to be pressurized. The MCFC could serve a niche market of data centers and hospitals. FuelCell Energy has recently made a commercial offering of MCFCs. These fuel cells will probably not have the same market penetration potential as SOFCs and thus would likely have little or no impact as a transition strategy for H2 use.
Direct Use of Hydrogen in Distributed Generation
Small gas turbines, less than 25 MW, can operate on H2 or H2-rich fuels with little or no modification, similar to gas turbines for central power generation. There have been some demonstrations of 5 and 10 MW systems with enriched H2 gas at refineries but not to the same extent as the demonstrations of large GE turbines for processing.9
INDUSTRIAL SECTOR
Industry is currently the largest producer and user of H2 in the United States (3 trillion ft3 of H2 annually—which represents less than 3 percent of the energy used by the sector). Steam reforming and water-gas-shift reactions and separations are the primary processes for hydrogen production; they are carried out in refineries and large-scale chemical plants. Natural gas is the primary feedstock for existing hydrogen production. Approximately 50 percent of the H2 consumed by industry is for ammonia production, 36 percent is for petroleum refining, 8 percent is for the production of methanol, and 6 percent is for other uses (DOE, 2003d).
Combustion offers potential for the industry-wide use of hydrogen. Industrial boilers and process heaters—fueled by the combustion of fossil fuels such as natural gas and coal—use 13.5 quadrillion Btu (quads), more than 75 percent of the total U.S. manufacturing energy. By 2050, the combined industrial energy demand is projected to be more than 26 quads. The use of hydrogen as a combustion fuel source for industrial boilers and process heaters offers the potential for a sizable end-use market for hydrogen—up to 2.6 quads of energy annually by 2050. In addition, there could be improvements in efficiencies—99 percent thermal efficiency versus 80 percent for conventional technology (DOE, 2003d). There is experience in the industrial sector using hydrogen blended with other fuels and diluents; there is little or no experience with H2-air and H2-O2 systems.
Systems studies, as well as conceptual designs and further investigations of component issues related to, for example, combustors, heat exchangers, and flue gas ducting, are needed in order to develop more fully the understanding of the role of H2 combustion technologies in the industrial sector.
SUMMARY OF RESEARCH, DEVELOPMENT, AND DEMONSTRATION CHALLENGES FOR FUEL CELLS
Despite great improvements in fuel cell technologies over the past decade and demonstration of promising performance, both stationary and automotive fuel cell systems still face large challenges. These primarily involve cost reduction: costs on the order of $500 to $800/kW-peak are required for competitive stationary systems, and costs on the order of $50 to $100/kW-peak are required for competitive FCVs. These cost levels are far below current levels for various fuel cell technologies that are in prototype and low-volume production. Additional challenges include fuel cell
durability: development goals are for 40,000 to 50,000 hours between major overhauls for stationary systems and 4,000 to 5,000 hours for automotive systems; the development of efficient and low-cost fuel reformers (see Chapter 8); and the development of vehicular hydrogen storage systems that are inexpensive, lightweight, compact, safe, and quick to refuel (see Chapter 4).
FINDINGS AND RECOMMENDATIONS
Finding 3-1. The federal government has been active in fuel cell research for roughly 40 years. Proton exchange membrane fuel cells (PEMFCs) applied to hydrogen vehicle systems are a relatively recent development (as of the late 1980s). The Department of Energy has spent more than $1.2 billion since 1978, and there has been considerable private sector investment for all fuel cell types. The DOE has spent $334 million since the 1980s on PEMFCs for transportation applications, most of it at national laboratories. Automakers and suppliers greatly expanded their PEMFC development efforts beginning in the later 1990s. In spite of the large federal and private sector investment, fuel cell prototype costs for light-duty vehicles are still a factor of 10 to 20 times too expensive and these fuel cells are short of required durability. Accordingly, the challenges of developing PEMFCs for automotive applications are large. Furthermore, the DOE’s near-term milestones for fuel cell vehicles appear unrealistically aggressive on the basis of the current state of knowledge with respect to fuel cell durability, storage systems, and overall costs. The choice of unrealistic targets can lead to programs that emphasize spending on extensions and expensive demonstrations of current technologies in lieu of breakthroughs that will probably be required if a fuel-cell-based hydrogen economy is to be realized. Industry is expanding its development; thus, the DOE should focus on fundamental research.
Recommendation 3-1a. Given that large improvements are still needed in fuel cell technology and given that industry is investing considerable funding in technology development, increased government funding on research and development should be dedicated to the research on breakthroughs in on-board storage systems, in fuel cell costs, and in materials for durability in order to attack known inhibitors to the high-volume production of fuel cell vehicles.
Recommendation 3-1b. Since a hydrogen transportation economy will probably not emerge without the development of reasonably priced, energy-efficient fuel cells, the transportation portion of the Department of Energy’s research, development, and demonstration program should emphasize fuel cells and their associated storage systems at the expense of “transition technologies” such as on-board reformers and hydrogen internal combustion engines. Since transition technologies mainly involve “development,” funding for these programs should be provided by industry. Of course, some component breakthrough technologies for reformation might be justified in supply-side programs, and the results might be applicable to on-board reformation.
Finding 3-2. Various fuel cell technologies are attractive for stationary applications. In fact, the major stationary fuel cell research, development, and demonstration programs—in particular, the solid oxide fuel cell and the molten carbonate fuel cell (neither of which requires hydrogen fuel)—are not part of the Department of Energy’s integrated direct-hydrogen program. Some private companies have committed to introducing proton exchange membrane stationary fuel cells without DOE funds, and these fuel cells appear to have applicability in a number of niche markets.
Recommendation 3-2. The Department of Energy should discontinue the proton exchange membrane (PEM) applied research and development program for stationary systems. The $7.5 million annual budget (FY 2003 and FY 2004 request) for that program could be applied to PEM fundamental and basic issues (exploratory research) for all applications.
Finding 3-3. During the past 20 years, a number of approaches have been used to encourage the application of alternative fuels and technologies in transportation and stationary systems. Most of these have failed because of the lack of real marketplace pull, shifts in government policies, and the relative disinterest of industry. The role of marketplace pull is especially important, as has been exhibited by the progress in batteries over the past decade to satisfy high-volume consumer electronics demand—for example, the rapid transition from nickel cadmium through nickel metal hydride to today’s lithium-ion battery packs.
Recommendation 3-3. As the Department of Energy develops its strategy for the hydrogen economy with respect to the role of public research, development, and demonstration policies, it should sponsor an independent study of lessons learned with respect to the lack of success and widespread market acceptance of previous alternative fuel technologies, as well as other technologies developed for transportation and stationary power systems. The purposes of this study would be as follows: (1) to assess the role of government policy and its stability as it affects industry and consumer behavior, (2) to affect strategies related to the introduction of hydrogen in the end-use sectors, and (3) to avoid repeating the mistakes of prior-technology-introduction programs, such as those for electric and natural gas vehicles and for phosphoric acid fuel cells for distributed generation. In addition, strengths and weaknesses of the Partnership for a New Generation of Vehicles Program and hybrid electric vehicle development should be analyzed, as the FreedomCar Program is structured for the development of fuel cell vehicles.
Finding 3-4. The role and use of hydrogen in stationary applications, such as in large-scale electric power production, in distributed electric generation, or for industrial applications, could be significant—before fuel cell vehicles are commercially viable as well as in the long term. The Committee on Alternatives and Strategies for Future Hydrogen Production and Use did not analyze the opportunities and trade-offs for stationary applications, especially vis-à-vis the transportation sector. Furthemore, as far as the committee can discern, and from reviewing the Department of Energy’s hydrogen research, development, and demonstration (RD&D) plan, the DOE has not developed a hydrogen RD&D strategy that systematically incorporates both the stationary and the transportation sectors, nor defined the various trade-offs and opportunities.
Recommendation 3-4. An independent, in-depth study, similar to the present study on the transportation sector, should be initiated to analyze the opportunities for hydrogen in stationary applications and to make recommendations related to a research, development, and demonstration strategy that incorporates considerations of both the transportation and the stationary sectors.