7
Carbon Capture and Storage
THE RATIONALE OF CARBON CAPTURE AND STORAGE FROM HYDROGEN PRODUCTION
Hydrogen is used extensively for its chemical properties, and on a global basis most hydrogen is produced from fossil fuels. As shown in Chapter 5, the committee believes that a transition to a hydrogen energy system would likely rely on hydrogen (H2) from the reforming of natural gas and from electrolysis powered by a grid mix inclusive of coal-fired power plants. The fossil resources combusted or used as feedstock during such a transition will produce by-product carbon dioxide (CO2). Thus, converting to a hydrogen economy based on fossil fuels would have no advantage in reducing CO2 emissions unless the CO2 can be isolated indefinitely.
Such a strategy is called carbon capture and storage (CCS).1 Figures 6-7 through 6-10 in Chapter 6 compare emissions of carbon, with and without carbon capture and storage, that would be associated with the fleet of U.S. light-duty vehicles as fuel cell vehicles (FCVs) penetrate the market over the period 2020 to 2050, replacing gasoline-fueled vehicles by 2050. Comparison of the curves in the year 2050 permits head-to-head comparison of projected carbon emissions that would be associated with fleets composed entirely of one power plant (that is, the conventional gasoline-fueled vehicle, gasoline hybrid electric vehicle [GHEV], or fuel cell vehicle) and, in the case of hydrogen, one of several possible production technologies. Even though the hydrogen fuel cell vehicle is assumed in the committee’s analysis to be 66 percent more efficient than GHEVs are, emissions in 2050 are almost the same when coal is the hydrogen source and CCS is not used versus GHEVs.
CCS may turn out to be one of the critical strategies for reducing net carbon emissions,2 or it may turn out to have shortcomings that limit its role. Work is under way worldwide to develop various separation and storage technologies, reduce projected costs, and understand risks.
Scale of Carbon Capture and Storage Associated with Hydrogen Production in a Hydrogen Economy
Table 7-1 presents the ratios of emitted carbon (C) to produced hydrogen used in this report for natural gas reforming and coal gasification, for both current and possible future technology cases (see Chapters 5 and 6 for details). Thus, for example, it is estimated that 5.1 kg C will be emitted to the atmosphere for each kilogram of hydrogen produced from coal gasification using current technology.
Hydrogen production from coal gasification results in about twice the carbon emissions of hydrogen production from the reforming of natural gas. CCS technology achieves a reduction of about 85 percent in atmospheric carbon emissions from either feedstock.
One of the main rationales for moving toward a hydrogen economy is to address the goal of improved environmental quality, including reduction in CO2 emissions. Carbon can be captured at central station production plants and stored, consistent with this goal. However, the quantity of carbon to be handled is enormous. (Capture is virtually impossible in vehicles burning gasoline or diesel fuel.) As discussed in Chapter 6, if hydrogen FCVs dominate the market in 2050, they will require the production of 100 million metric tons
TABLE 7-1 Estimated Carbon Emissions as Carbon Dioxide Associated with Central Station Hydrogen Production from Natural Gas and Coal
State of Technology Development |
Technology |
Ratioa Without Carbon Capture and Storage |
Ratioa With Carbon Capture and Storage |
Current |
Natural gas reforming |
2.51 |
0.42 |
|
Coal gasification |
5.12 |
0.82 |
Possible future |
Natural gas reforming |
2.39 |
0.35 |
|
Coal gasification |
4.56 |
0.60 |
aMass of carbon emitted divided by mass of hydrogen produced. |
(Mt) of hydrogen per year.3 Assuming possible future technologies, without CCS, the associated annual carbon emissions from natural gas production would be 255 Mt C, and 518 Mt C from coal plants. CCS technology reduces emissions to 42 Mt C and 83 Mt C for natural gas and coal, respectively (less than 100 percent of the CO2 will be removed by any competitive technology). Thus, the scale of the task of capturing and storing most of the CO2 associated with an all-hydrogen U.S. fleet of light-duty vehicles in 2050 could be approximately 200 to 400 Mt C/yr (assuming insignificant hydrogen production from nuclear or renewable energy sources).
Today’s annual U.S. CO2 emissions from light-duty vehicles, about 320 Mt C, are about 20 percent of U.S. CO2 emissions and 5 percent of global emissions from all sources. It was beyond the scope of this report to generalize beyond transportation to the role of CCS in hydrogen production for use in other sectors of the U.S. economy (hydrogen use in industry and in buildings, for example).
Capturing and storing 200 to 400 Mt C annually by 2050 is a huge task. On the order of a thousand projects the size of the first two CO2 geological storage demonstration projects (discussed below, in the subsection “CCS Demonstration Projects”) would be required. These projects each capture and store about 300,000 metric tons C/yr. This is also the scale of CCS planned for the proposed FutureGen Project (see below).
Carbon Emissions Associated with Current Hydrogen Production
At the present time, global crude hydrogen production relies almost exclusively on processes that extract hydrogen from fossil fuel feedstock (see Figure 7-1). It is not current practice to capture and store the by-product CO2 that results
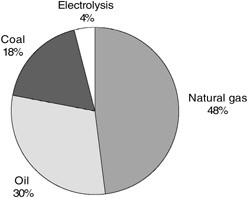
FIGURE 7-1 Feedstocks used in the current global production of hydrogen. SOURCE: Courtesy of Air Products and Chemicals (2003).
from the production of hydrogen from these feedstocks. Consequently, more than 100 Mt C/yr are vented to the atmosphere as part of the global production of roughly 38 Mt of hydrogen per year.4
Some but not all of the CO2 is emitted in concentrated streams at elevated pressure. At an intermediate step in the production of hydrogen from fossil fuels, there is a mixture of CO2 and H2 under pressure, together with impurities. At this stage, there are two options:
-
Extract CO2 at high purity and elevated pressure, which is well matched to CCS technology. Left behind is a less-than-pure hydrogen, but it is of high enough concentration to be commercially useful. Such hydrogen is sometimes called refinery hydrogen. It is suitable for applications such as hydrogen-fueled internal combustion engines, but some CO2 and other impurities will be emitted.
-
Extract hydrogen at high purity; such hydrogen is sometimes called fuel-cell-grade hydrogen. The residue still contains some hydrogen, which can be burned to produce steam or electricity. If the residue is burned in air, the exhaust stream contains the CO2 from the conversion, but also a large fraction of nitrogen from the atmosphere. Then, the CO2 is dilute and more difficult to capture than in option 1.
There is a recent trend in refineries away from the first option and toward the second, because of the falling cost of hydrogen separation from gas mixtures via pressure-swing absorption. If the CO2 from refinery production of hydrogen
is to be captured and stored, this trend will complicate the process. Similarly, fuel cells require high-purity hydrogen. Plants built to produce this hydrogen and to capture and sequester CO2 will require equipment and processing not used in current plants. The joint goals of high-purity hydrogen and low-cost CCS may demand new capture technology.
CCS Demonstration Projects
Two CCS demonstration projects are currently under way, one in Norway and one in Canada:
-
At the Sleipner gas field in the North Sea off Norway, natural gas is produced containing about 10 percent CO2. The CO2 concentration exceeds the concentration allowed in the European natural gas grid by about a factor of 4. Normally in such cases, CO2 is stripped from the gas onshore and vented to the atmosphere. Since 1996, motivated by a carbon tax of about $140/t C (in U.S. dollars), the field operators are stripping CO2 offshore and injecting it into the nearby Utsira formation, a very large saline aquifer that does not contain hydrocarbons.
-
At the Weyburn oil fields in Saskatchewan, Canada, CO2 captured at a coal-to-methane plant in North Dakota and piped across the border is being injected, with the joint objective of enhanced oil recovery (EOR) and CO2 storage.
Both projects store about 0.3 Mt C/yr.
Several additional CCS demonstration projects are being planned at roughly the same scale. Notable among them is the FutureGen Project, proposed in the spring of 2003 by the Bush administration. In this project, a 275 megawatt (MW) coal-fired power plant would produce both electricity and hydrogen and would capture CO2 for offsite storage. Announced at the same time and coupled with FutureGen is the U.S. Carbon Sequestration Leadership Forum, aimed at developing international partnerships for the commercialization of CCS technology.
In addition to demonstration projects, experience bearing on CCS technologies is being gained through EOR projects that inject CO2 into partially depleted oil fields. The first EOR project was in West Texas in 1972, and most of the EOR in the world today is still concentrated there.
Only about one-quarter of the CO2 used in EOR is derived from industrial sources (Hill, 2003, p. 25). Most is extracted from natural CO2 formations. The value of CO2 for EOR has been sufficient to warrant the construction of several multi-hundred-kilometer (km) CO2 pipelines, including one of 800 km from the McElmo Dome in southwest Colorado to the Permian basin oil fields in West Texas.
Until recently, EOR was not thought of as a carbon storage strategy. Once the CO2 has done its work and production is concluded, EOR project managers have not considered whether the CO2 would remain belowground for decades, centuries, or millennia. Joint optimization of EOR and long-term CO2 storage could lead to revisions in EOR practices. Thus, EOR to date provides only partial precedents for CCS.
Capture
Hydrogen production involves the transfer of most of the energy from the feedstock chemical compound to the product molecular hydrogen. Adding the objective of CO2 capture complicates the design of the equipment and increases the costs of production. However, most of the plant components required to capture CO2 are already required to produce hydrogen, so the fractional increment in the cost of hydrogen owing to CO2 capture is not large: in the committee’s estimates (see below), between 10 and 20 percent for natural gas and less than 10 percent for coal. The fractional increment is substantially smaller than would be incurred if CO2 capture were added to electricity production.
An intriguing concept is that of the co-capture and co-storage of impurities with the CO2, saving the costs currently incurred to prevent these impurities from becoming air or water pollutants. For example, sulfur in coal can be co-captured with the CO2 (as either hydrogen sulfide [H2S] or sulfur dioxide [SO2], depending on the plant configuration), piped with the CO2 to a storage location, and co-stored as a single fluid.
Table 7-2 shows estimated hydrogen production costs for large-scale plants.5 The “with carbon capture and storage” cases also show the estimated costs of storage. Both sets show an imputed cost of CO2 emissions on a $50 per metric ton (tonne) C basis.6
The estimated cost of carbon associated with either type of plant—natural gas or coal—is calculated as the sum of storage costs plus capture costs: the storage costs are shown in Table 7-2, and capture costs are defined as the difference between the plant production costs with and without CCS. It is interesting to note that the assumed cost of carbon emissions is insufficient to justify CCS for natural gas plants, whereas it approximately balances the additional costs for CCS in a coal plant. The capture costs for coal are a smaller percentage of the plant costs for the technologies assumed in this report (see Chapter 8); for example, the CO2 in this study’s coal gasification plant is available for capture at a higher partial pressure, which reduces the cost of capture. The capture costs, as a percentage of the cost of production at the plant without CCS, are 18 percent and 11 percent for
5 |
The scale of this study’s large plants is 1200 t H2/day, or 2.0 GW H2 (higher heating value), or 1.7 GW H2 (lower heating value). |
6 |
The fuel costs assumed (see Chapter 5 and Appendix E) are as follows: $4.50 per million Btu (higher heating value) for natural gas; $1.22 per million Btu (higher heating value) for coal; and 4.5 cents/kWh for electricity. The cost of storage is highly uncertain at this time and has not been a focus of this committee’s analysis. The committee assumed $37/t C, which is consistent with the range of current estimates. The imputed cost of CO2 emissions is even more uncertain. The $50/t C cost arbitrarily chosen in this report is a point of departure for many analyses (see Chapter 5). |
TABLE 7-2 Estimated Plant Production Costs and Associated Outside-Plant Carbon Costs (in dollars per kilogram of hydrogen) for Central Station Hydrogen Production from Natural Gas and Coal
|
|
Without Carbon Capture and Storage ($/kg H2) |
With Carbon Capture and Storage ($/kg H2) |
|||
State of Technology Development |
Energy Source |
Plant |
Carbon Emissions at $50/t C |
Plant |
Storage Costs at $37/t C |
Carbon Emissions at $50/t C |
Current |
Natural gas |
1.03 |
0.13 |
1.22 |
0.09 |
0.02 |
|
Coal |
0.96 |
0.26 |
1.03 |
0.16 |
0.04 |
Possible future |
Natural gas |
0.92 |
0.12 |
1.02 |
0.08 |
0.02 |
|
Coal |
0.71 |
0.23 |
0.77 |
0.15 |
0.03 |
natural gas, current and possible future cases, respectively, and they are 8 percent for coal, both current and possible future cases. The storage costs, on the other hand, are roughly twice as large for coal as for natural gas, because roughly twice as much CO2 must be stored per unit of hydrogen produced. As a result, the capture costs are comparable to the storage costs for natural gas, but they are much less than the storage costs for coal.
It is interesting to express carbon costs in various units. The cost of storage, for example, at $10 per metric ton of carbon dioxide, or $37 per metric ton of carbon, is approximately as follows (assumptions are in footnotes):
-
9 cents per gallon of gasoline,7
-
$4 per barrel of oil,8
-
$24 per U.S. ton of coal,9
-
54 cents per 1000 standard cubic feet (scf) of natural gas,10
-
0.8 cent per kWh of electricity from coal,11
-
0.4 cent per kWh of electricity from natural gas,12
-
18 cents/kg H2 from coal,13
-
9 cents/kg H2 from natural gas,14 and
-
$220 billion per year implicit flow through the global energy system.15
Storage
After CO2 leaves the plant gate, it can be directed to several kinds of destinations where it can be stored in several chemical forms. The destinations that have received most attention are geological strata deep belowground and the deep ocean. Both destinations may have storage capacity for a century of aggressive global carbon management. Among the other concepts are storage as stable carbonates (produced by the exothermic reaction of CO2 with certain magnesium and calcium minerals) and storage as elemental carbon (charcoal)16—in both cases, presumably, piled aboveground.
It is often suggested that CO2 should not be stored but somehow recycled. Indeed, there are commercial uses for CO2 in industrial process cleaning, in dry ice, in carbonated beverages, and elsewhere in the economy, but these typically delay the release of CO2 to the atmosphere by days or weeks, so recycling is not an alternative to CO2 storage. It is also possible to convert CO2 to hydrocarbons or other carbon-containing compounds, but significant energy would be required (producing more CO2), and few uses of these compounds will keep the carbon from the atmosphere for as long as a year. Once carbon is brought out of the ground in fossil fuels, the industrial economy provides very few incidental routes to long-term storage; exceptions include asphalt road-beds and shingles. Only deliberate storage can prevent fossil fuel carbon from becoming atmospheric CO2.
As seen in Table 7-2, if the total CO2 storage cost is as little as $37/tonne C, it is a small fraction of the cost of H2 produced from fossil fuels at large scale. This estimate was derived from the cost of pipelines and wells. It is possible, however, that other costs will dominate, such as those of permitting and monitoring (see below).
Infrastructure
The first step in CO2 storage is transporting the CO2 from the production site to the storage site. An entirely new infrastructure will be required for this purpose, presumably a
pipeline system with trunk lines and branch lines. There is already considerable experience with CO2 pipelines for EOR, but institutional issues, including financing, construction, operation, and monitoring of the infrastructure, may be quite different when the goal is storage.
In a recent analysis of the cost of a large CO2 pipeline leading to a disposal well 100 km from a large coal-to-hydrogen plant, the pipeline itself is found to contribute $13/t C (Ogden, 2003). This is one-third of this study’s assumed total storage cost. At 5 t C/t H2, a mass ratio appropriate for coal (see Table 7-1), this cost is equivalent to 6.5 cents/kg H2.
Because of economies of scale in pipelines, the costs per unit of CO2 stored or per unit of hydrogen generated increase as the scale of the hydrogen production system decreases. Unit costs vary approximately as the inverse square root of the flow rate. The pipeline discussed here has a capacity of 10,000 t CO2/day; roughly two of these large pipelines are required to handle the CO2 from this study’s central station coal plant. Midsize production of hydrogen from natural gas also may turn out to be compatible with CCS. However, it is very likely that if hydrogen is generated in the distributed natural gas systems modeled in this report, the costs of storage (and probably capture as well) will be prohibitive. The dividing line is not clear: further work is required to understand the size of the smallest hydrogen production plant at which CCS is economically feasible. Unless unexpected breakthroughs in technology are realized, however, small-scale production of hydrogen from natural gas will be consistent with the objective of climate change only if it is considered a stepping-stone to production strategies that limit emissions of CO2.
Geological Storage
Storage belowground can be in sedimentary formations that are either hydrocarbon bearing or largely free of hydrocarbons (“brine formations”). Hydrocarbon-bearing formations include oil and gas reservoirs and coal seams. Thanks to experience with EOR, much has been learned about migration of supercritical CO2 as a distinct phase, dissolution into hydrocarbons and brine, and chemical interaction with host rock. EOR probably provides the lowest-cost early opportunities for CO2 storage in many areas of the world. Storage can also be in reservoirs that were once sources of commercial hydrocarbons but that are no longer productive; CO2 injection would not offer any economic benefits, but, overall, these destinations may offer more total storage capacity than EOR sites do, with comparable permanence of storage.
Brine formations appear to contain much larger storage capacity than do hydrocarbon reservoirs. The Sleipner project referred to above is an example of such storage. Hydrocarbon reservoirs have held buoyant fluids for geological epochs; brine formations have no such track record. Accordingly, the durability of storage in brine formations is under intense investigation at present.
In geological storage, the CO2 is injected as a supercritical fluid, with roughly half the density of water.17 To achieve the necessary supercritical pressures, CO2 is injected at high pressure at least 800 m below the surface.
Deep-Ocean Storage
The storage of CO2 in the deep ocean is an area of active modeling and some experiments. At issue is the retention time for various sites as well as the biological impacts. Advocates of deep injection point out that even in the absence of deliberate injection, the oceans already receive a portion of the carbon released from fossil fuels because of the continuous exchange of CO2 between the atmosphere and the oceans. Add CO2 to the atmosphere and some of it will move naturally to the ocean, as equilibrium is sought at the ocean surface.18 Also under study are the biological impacts of additional CO2 in the near-surface ocean—for example, the impacts on coral reefs.
Storage in Biological Carbon
Biological strategies to remove CO2 from the atmosphere, although not the focus of this report, deserve mention. These strategies remove carbon from the atmosphere by photosynthesis. On land, storage usually takes place at the same site as that of capture—for example, in a tree. Increases in atmospheric CO2 enhance plant growth up to a point that is yet to be fully understood. In the ocean, capture is via various organisms at the surface that then sink into the deep ocean.
The initial costs of biological carbon sequestration on land are small, and joint gains from land improvement plus carbon storage may be available. However, the earth’s capacity for biological carbon sequestration is limited, particularly for long-term storage (many decades or centuries), which could conflict with food production and other uses of the land. The mass of carbon aboveground in terrestrial vegetation is roughly equal to the mass of carbon in the atmosphere, about 700 billion tons of carbon (IPCC, 2001a). Imagine the future production of 700 billion tons of carbon from fossil fuel resources belowground, and imagine that the CO2 is to be captured in forests and grasslands instead of building up in the atmosphere. The result would be to double the biological carbon stock. Such a huge change is likely to be inconsistent with the retention of ecosystem quality. Local environmental impacts of biological carbon storage also need to be considered.
The Carbon Storage Market
In a carbon management regime designed to mitigate climate change, a value will be put on not emitting carbon into the atmosphere. Those capable of storing carbon will compete for the opportunity to do so, creating a CO2 storage market. Low-cost storage will be available at only a few facilities in only a few locations; storage at higher cost will be available at many more locations. Owners of low-cost storage capacity will be at a commercial advantage. As the demand for storage grows, the clearing price for storage may climb as a result of greater demand or fall as providers accumulate knowledge that translates into lower costs.
The lowest-cost storage is likely to be at EOR sites. Today, the oil industry pays about $10 to $15/t CO2 (roughly, $40 to $60/t C) for CO2 delivered to the EOR site (Kuuskraa and Pekot, 2003). In the United States in 2001, about 10 Mt C/yr were injected for EOR, enhancing domestic oil production by 180,000 barrels per day (bbl/d) (Hill, 2003), or about 2 barrels of oil produced for each metric ton of CO2 injected.
The next-lowest-cost storage is likely to be at depleted oil and gas fields, where reservoir geology is already known, wells suitable for injection of CO2 may have already been drilled, relevant permits may already exist, and subsurface rights may be well defined.
Those purchasing CO2 storage will take into account the proximity and capacity of the storage site. Buyers and sellers will allocate costs for site characterization, leakage monitoring, and liability insurance.
Storage sites will differ in many respects. The value of a storage site may increase when the site can be demonstrated with high probability to be effective for a long time, when the loss of storage integrity can be easily detected, and when damage from the loss of storage integrity is small. One can imagine storage sites rated much as bonds are rated, with lower-quality storage being valued less.
The supplier of carbon storage may be able to gain further revenue by providing additional services—for example, offering co-storage of carbon and sulfur, relieving the purchaser of storage of aboveground sulfur management costs.19
Permitting
A CO2 storage regime can emerge only if public acceptance of the concept is widespread. Among the critical issues are these (Socolow, 2003):
-
Trust. Public trust is critical. To what extent will openness, lack of bias, fairness, and vigilance be achieved?
-
Goals. What constitutes success? Will society be relaxed about the loss of 1 percent of the stored CO2 each year through slow leaks? What about the loss of 1 percent per year from 10 percent of the sites?
-
Permissiveness. The level of leakage allowed during the first few decades of storage can probably be greater than that allowed in later decades, not only because experience will permit improvements, but also because the total quantities stored will increase over time. How can the permitting process be made sufficiently permissive?
-
Reversibility. Should the storage system be one that future generations can undo?
-
Storage integrity at individual sites. Concentrations of more than a few percent of CO2 in air are dangerous, so bulk releases of CO2 must be guarded against. Under some conditions, safety may be an issue, as evidenced by the lakes in Africa that erupted with CO2, asphyxiating many local residents.20 Upward migration of injected CO2 could contaminate hydrocarbon reservoirs or surface drinking water supplies, so certain slow releases may also be of concern. How should such risks be addressed?
-
Property rights to storage space. Are ownership rights belowground clear? What about below the ocean floor and in the ocean? The market in carbon storage will generate requirements for well-defined property rights, attribution of ownership, liability rules, insurance, monitoring, and, in some cases, active intervention to limit damage.
-
Net carbon. It will be important to quantify, for various technologies and energy supply systems, the additional carbon that will be brought out of the ground to provide the energy necessary to capture and store the CO2.
-
Monitoring. Can infrastructure and storage be designed in ways that facilitate attribution and monitoring (e.g., by adding tracers to the injected gas)? What techniques are available to respond constructively to evidence that stored materials are not behaving as expected? How can long-term monitoring be institutionalized?
-
Precedents. There are two obvious precedents in the United States for the storage of CO2: underground injection of hazardous waste and nuclear waste storage. Both offer lessons to the designers of CO2 storage. The underground injection of hazardous wastes is governed by an Environmental Protection Agency (EPA)-regulated permitting process based on detailed modeling intended to prove that nothing serious will happen belowground after injection, followed by little, if any, post-injection monitoring and verification of what is actually happening belowground. The program to store nuclear waste began with promises of leakproof, very
19 |
Today, in Alberta, Canada, H2S and CO2 are routinely removed from natural gas between wellhead and transmission pipeline and then co-stored belowground. |
20 |
For example, in 1986, Lake Nyos in Cameroon erupted in a massive outgassing of CO2, killing 1800 people nearby. The cold bottom water of the lake, saturated with CO2 seeping up from the earth below, became unstable. The CO2-laden air, heavier than ordinary air, filled nearby valleys. Further information is available online at http://helios.physics.uoguelph.ca/summer/scor/articles/scor158.htm (accessed December 11, 2003) and http://perso.wanadoo.fr/mhalb/nyos/index.htm (accessed December 11, 2003). |
-
long term storage. Skepticism that such promises could be kept has severely hampered progress.
FINDINGS AND RECOMMENDATIONS
Finding 7-1. It is highly likely that fossil fuels will be the principal sources of hydrogen for several decades. It follows that an expanded role for hydrogen will have a much larger positive impact on the mitigation of climate change if carbon capture and storage technologies are successfully integrated into the fossil fuel production of hydrogen.
The majority of the early carbon capture and storage projects might not involve hydrogen, but could instead involve the capture of the CO2 impurities in natural gas, the capture of CO2 produced at electric plants, or the capture of CO2 at ammonia and synfuels plants. All of these routes to capture, however, share carbon storage as a common element, and it is carbon storage that raises the most difficult institutional issues and issues of public acceptance.
As of 2002, for the first time, the Department of Energy’s programs related to carbon sequestration are listed as an associated program of its hydrogen program.
Recommendation 7-1. The U.S. Department of Energy’s hydrogen program needs to be well integrated with the carbon capture and storage program, to assure that any expanded role for hydrogen produced from fossil fuel has a positive impact on the mitigation of climate change. Such integration will enable the hydrogen program to identify critical technologies and research areas that can enable hydrogen production from fossil fuels with CO2 capture and storage.
Tightening the coupling of the two programs should facilitate setting priorities in those portions of the hydrogen program addressing hydrogen production from fossil fuels and biomass. It should also promote the exploration of overlapping issues—for example, the co-capture and co-storage with carbon dioxide of pollutants such as sulfur during hydrogen production.
Because of the hydrogen program’s large stake in the successful launching of carbon capture and storage activity, the hydrogen program should participate in all of the early carbon capture and storage projects, even those that do not directly involve carbon capture during hydrogen production. These projects will address the most difficult institutional issues and the challenges related to issues of public acceptance, which have the potential of delaying the introduction of hydrogen in the marketplace.
Finding 7-2. The Department of Energy’s recently announced FutureGen Project is intended to demonstrate the production of hydrogen and electricity from coal at a large scale, while capturing CO2. FutureGen may become the world’s earliest carbon capture and storage project integrated with hydrogen production. This project should provide an opportunity to integrate the development of advanced technologies for the production of hydrogen with CO2 capture and storage.
Recommendation 7-2. The FutureGen Project should be managed to encourage the development of technologies that integrate hydrogen production with carbon dioxide capture. FutureGen should have strong research and development components.
Finding 7-3. The successful integration of carbon capture and storage will depend at least as much on institutional factors and public acceptance as on engineering prowess and geological opportunities. Institutional factors include property rights at storage sites, the management of infrastructure, insurance and liability, and the funding of monitoring and verification, including those efforts over the very long term. Public acceptance will depend on achieving and maintaining trust, which will require processes that are regarded as open and fair. The public discussion of local environmental and health risks in this realm has hardly begun, nor has public discussion regarding criteria for long-term storage, such as criteria for durability and verifiability. It may be possible and desirable to achieve broad consensus that early criteria are more permissive and later ones are tougher.
Recommendation 7-3. The Department of Energy should foster public discussion of institutional factors affecting carbon capture and storage, including property rights at storage sites, the management of infrastructure, insurance and liability, and the funding of monitoring and verification, including those efforts over the very long term.
The DOE should foster the identification of the issues likely to have the greatest impact on public acceptance of carbon capture and storage. It should encourage public discussion of local environmental and health risks. It should encourage public discussion of what constitutes “adequate” storage, from the standpoint of durability and verifiability. It should explore the merits of broad agreement that early criteria be more permissive and later ones tougher.